Muscle
Mass (g)
Muscle length (cm)
Lf (cm)
Lf/Lm
Ls (μm)
Sn
Pennation angle (°)
PCSA (cm2)
Supraspinatus
34.0 ± 4.3a
8.5 ± 0.4a
4.50 ± 0.32a
0.53 ± 0.03b
3.23 ± 0.05b,c
16,655 ± 1182a
5.1 ± 0.8a
6.65 ± 0.56a
Infraspinatus
78 ± 7.5a
2.1 ± 0.5c,d
6.57 ± 0.33d
0.55 ± 0.02b
3.18 ± 0.06§ b
24,332 ± 1203d
1.4 ± 0.4† II
10.71 ± 0.95a
Teres minor
21.2 ± 2a
10.8 ± 0.6d,e
6.09 ± 0.35d
0.57 ± 0.03b
2.80 ± 0.07d,e
22,569 ± 1299d
0.6 ± 0.3d
3.18 ± 0.30a
Subscapularis
101.8 ± 11.5a
13 ± 0.6d
6 ± 0.47d
0.45 ± 0.02a
2.52 ± 0.09d, e
22,069 ± 1735d
0 ± 0d,e
15.53 ± 1.41a
The average muscle fiber length has been reported to be between 4.5 and 6.6 cm, with the supraspinatus muscle showing the smallest fiber length (Table 21.1) [2]. To compensate for the small fiber length, the supraspinatus muscle has been shown to operate over the widest range of sarcomere lengths [2].
21.2.2.2 Pennation Angle
The pennation angle is defined as the angle between the muscle fibers and the intramuscular central tendon. For mechanical and geometrical reasons, pennated muscles work better when the pennation angle is smaller.
The pennation angle in the intact supraspinatus muscle varies from 10° for the medial fibers to 85° for the lateral fibers [3–5]. This provides greater contraction ability on the medial side, with a subsequent increased shear stress on the tendon when compared to the lateral side. Meyer et al. [6] reported an increase in the pennation angle after rotator cuff tears. Others found a positive correlation between the pennation angle of the supraspinatus muscle and the tear size of the supraspinatus enthesis [7].
21.3 Pathophysiology of the Rotator Cuff Muscle
Although rotator cuff tears are a common pathology, the lesion rarely involves directly the muscular belly or the muscle-tendon junction. Nevertheless, muscles are deeply and substantially involved through three mechanisms: retraction, atrophy, and fatty infiltration. These pathologic changes may lead to worse outcomes and may guide surgical indications.
21.3.1 Retraction and Atrophy
A rotator cuff muscle without stimulation and with a torn tendon will undergo atrophy and retraction. Ruptures lead to severe changes in the muscle [8–10]. These changes are more accentuated in slow contracting type 1 muscle fibers when compared to fast contracting type 2A and 2B fibers [6, 11]. Fibrous tissue is stored intramuscularly [11], and a short time after the occurrence of the tear, the muscle starts to retract and loses its ability to build up tension [12]. In a supraspinatus tenotomy model in sheep, the muscle retracted 29 mm on average 16 weeks after tenotomy. This retraction corresponds with the physiological range of movement of the muscle. The pennation angle increased from 30° to 55°, and the cross-sectional area decreased by 57 % compared to the healthy opposite side [12]. This retraction leads to shortening of the muscle fibers by the breakdown of serially arranged sarcomeres up to 50 %. Due to this loss of sarcomeres, the muscle gets shorter instead of thinner.
Over the years, the cross-sectional area can decrease. With increasing retraction, the pennation angle of the muscle increases. As discussed above, this leads to the incorporation of fatty infiltration in between the muscle fibers. In this model, retraction and atrophy are therefore caused by shortening of “healthy” muscle tissue and not muscle degeneration in the proper sense [6]. Steinbacher et al. [13] confirmed this in a study where they reported that the cause for atrophy in rotator cuff tears greater than or equal to 3 cm (Bateman grade III and IV) is found in the decrease of the absolute myofibril volume and not in the death of fibers [13].
21.3.1.1 Changes in Gene Expression with Atrophy
Gene expressions of several genes that induce muscle atrophy are altered after rotator cuff tears. The two key regulators for the induction of Muscle RING-finger protein-1 (MuRF1) und Atrogin-1 are upregulated shortly after the rotator cuff tear [14, 15]. In massive rotator cuff tears, genes that are involved in sustaining muscle atrophy (e.g., Forkhead box protein O1A (FOXO1A), Calpain, Ubiquitin-conjugating enzyme-E2B (UBE2B) and -E3A (UBE3A), and Cathepsin B (CTSB)) are greatly upregulated when compared to smaller tears. This could explain the fact that rotator cuff reconstructions show a better outcome after small tears compared to massive tears.
21.3.1.2 Generation of Force in Atrophic Muscle
An increased pennation angle results in an inefficient force application from the muscle fibers to the tendon due to the fact that the muscle fibers are not pulling in the direction of the tendon [9].
Meyer et al. [16] reported in a study, in which they performed a tenotomy of the infraspinatus tendon in sheep, that the fatty infiltration negatively correlates with the loss of strength and that atrophy correlates with the contractile amplitude. The larger the muscle density and the smaller the fatty infiltration measured in computer tomography [16], the greater is the maximal force of the muscle. The maximum contraction force reached by the muscle decreased significantly with increasing atrophy. This finding is crucial for the outcome of a reconstruction. Even if a rotator cuff tendon is adapted without traction to the footprint, its force may be diminished. Furthermore, passive tension is increased, which may lead to a limitation of the range of motion.
21.3.2 Fatty Infiltration
Deposition of fat into the muscle is termed fatty infiltration (Fig. 21.1). Fatty infiltration is a common finding not only in cuff tears but also in neurologic lesions of the rotator cuff. This infiltration can occur within different sites of the muscle, for example, in the interstitial space [13] where its accumulation leads to a limitation of its mechanics [6, 17]. Its accumulation not only occurs in between the muscle fibers but also in type I muscle fibers in the sarcoplasm [13]. On the other hand, fatty infiltration is also found in the extramuscular space (epimysium of the muscle belly) as well as in the torn tendon [10].
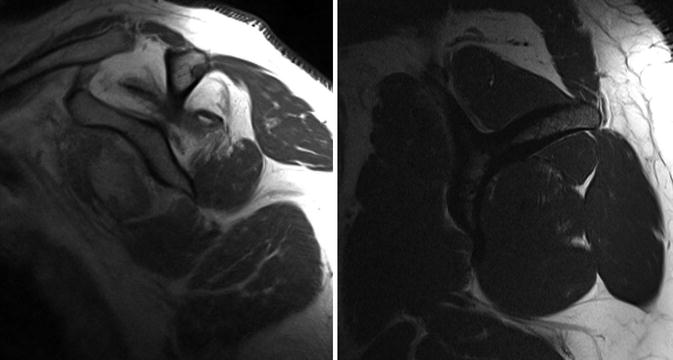
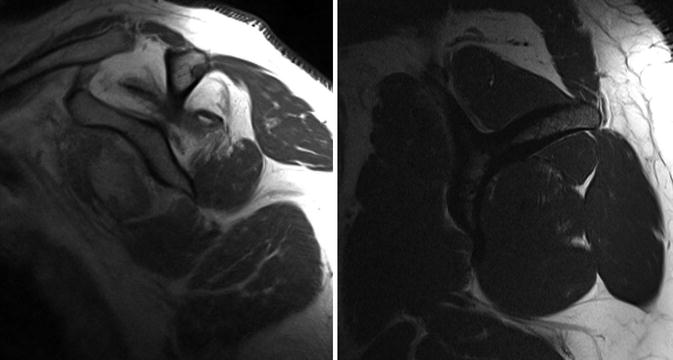
Fig. 21.1
(a) Sagittal MR scans of the shoulder showing fatty infiltration Goutallier stage 4 and (b) sagittal MR scan of the shoulder showing fatty infiltration Goutallier stage 1
Over the years, different theories were established to explain the origin of the fatty infiltration.
21.3.2.1 Changes in Muscle Architecture Lead to Fatty Infiltration
Rotator cuff tears and the associated loss of tension seem to favor processes that induce fatty infiltration [18]. After a rotator cuff tear, muscle fibers shorten and the pennation angle increases [6]. This leads to a newly created space, which is filled with fat and connective tissue (Fig. 21.2). This fatty infiltration in between the muscle fibers decreases the elasticity of the muscle, leading to decreased mechanical properties of the muscle. This theory is supported by the fact that the fatty infiltration correlates with the size of the rotator cuff tear [19, 20]. Furthermore, the progression of the fatty infiltration is inversely proportionate to the strength development [21]. This indicates that the loss of strength is not only caused by the atrophy but also by the fatty infiltration [9].
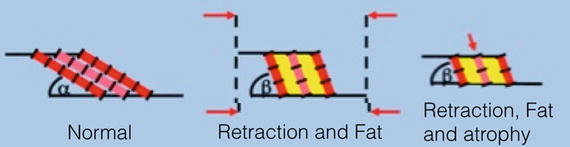
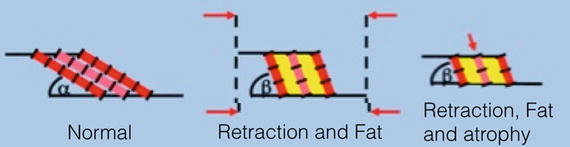
Fig. 21.2
(a) Normal muscle. (b) Retraction of muscle (decrease of x) leads to an increased pennation angle (beta) and to newly created space, which is filled with fat (yellow) (c) Atrophy (decrease of y) leads to a decrease in cross sectional area
21.3.2.2 Changes in Gene Expression Patterns Lead to Fatty Infiltration
Frey et al. [22] were able to show that after tenotomy of the infraspinatus muscle in sheep, several transcription factors that are important for myogenic differentiation are upregulated. This suggests that the body intends to solve the problem by increasing the amount of muscle. On the other hand, CAAT/enhancer binding protein β (C/EBPβ) and peroxisome proliferator-activated receptor γ (PPARγ), two factors involved in adipogenesis, were upregulated. The concentration of these factors significantly decreased after refixation of the rotator cuff.
21.3.2.3 Traumatic Partial Denervation May Lead to Fatty Infiltration
Another possible cause for the development of fatty infiltration may be related to neurogenic changes. This is supported by the fact that 7 of 28 patients with a complete rupture of the rotator cuff showed signs of a peripheral neuropathy in electromyography [23]. Albritton et al. [24] reported a partial denervation after complete tear of the supraspinatus tendon. This may lead to atrophy not only of the supraspinatus but also of the infraspinatus muscle [24].
The fact that neurogenic injuries lead to a fat distribution pattern that is more diffuse and irregular when compared to the one seen after rotator cuff tears makes it unlikely that denervation is the main factor contributing to fatty infiltration.
21.3.2.4 Increased Neovascularization and Amount of Mitochondria Correlate with Fatty Infiltration
Gerber et al. [17] were able to show that an increased neovascularization of the supraspinatus muscle and an increase in the amount of mitochondria occur after rotator cuff tears in the human. This correlated with the fatty infiltration and atrophy [17].
In another study [25], an increase in two angiogenetic factors (hypoxia-inducible factor (HIF) and vascular endothelial growth factor (VEGF)) were shown after rotator cuff tears.
21.4 Tendon and Enthesis
21.4.1 Ultrastructure and Physiology of the Tendon
Tendons of the rotator cuff are essentially made of two components: an extracellular matrix (ECM) and a cellular component. The extracellular matrix, which is the most important part of tendons in terms of mechanical properties, is made up of collagen (65–80 %), elastin (1–2 %), and a mixture of water, proteoglycans, glycosaminoglycans (GAGs), and glycoproteins. In rotator cuff tendons, there are more proteoglycans than in other purely tensional tendons. The most common proteoglycans are called decorin and biglycan (containing one or two GAG chains).
The cellular component of the tendon is divided into tenoblasts and tenocytes, representing 95 % of the whole amount of cells. Tenoblasts are precursors of tenocytes [26], while tenocytes are mature cells producing collagen and other components of the ECM.
The collagen fibers of the tendon are directly responsible for its mechanical properties. Lake et al. [27, 28] have extensively investigated the ultrastructure and mechanical properties of the rotator cuff tendons, especially of the supraspinatus tendon in recent works. The shoulder joint has a great range of motion and subsequently experiences multiaxial stresses. This is reflected in the collagen pattern having different distributions in tendon tissue. The stress-strain curve of the supraspinatus tendon, the most complex and commonly involved in damage, shows particular mechanical properties. These properties are different in the anterior, posterior, and bursal joint sides and can be attributed to the varying distribution of collagen. While axial loading shows higher stiffness in medial and anterolateral parts, resistance to transverse loading results in higher stiffness in posterolateral and anterolateral sides. This may be explained by the multidirectional complex loadings near the osteotendinous insertion.
21.4.2 Ultrastructure and Physiology of the Enthesis
The enthesis is the intermediary between the tendon and bone, and it has a remarkable difference in mechanical properties. The enthesis exists as a means of reducing the concentration of stress between the soft tendinous tissue and the hard bony tissue. As such, this site is most prone to rupture because of the great stresses it has to experience. The healthy enthesis employs different strategies to increase the strength of this crucial zone such as functional grading and change in microstructure through a transitional tissue, a reduced angle of fiber direction at the attachment, and interdigitation of tissue with bone [29].
According to literature, the enthesis is commonly described as consisting of four distinct zones (Fig. 21.3). More recent literature suggests that in reality these four zones are not distinctly segregated; rather, it exists as a fibrocartilaginous zone and has a graded transition from tendon to bone [30]. These four zones have been described as follows:
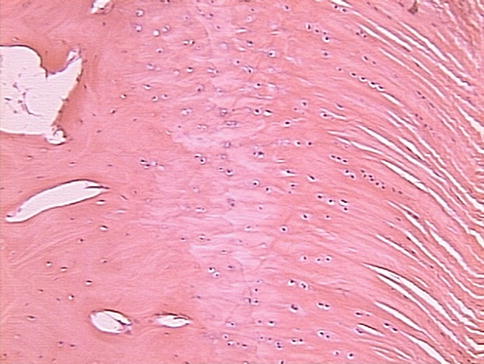
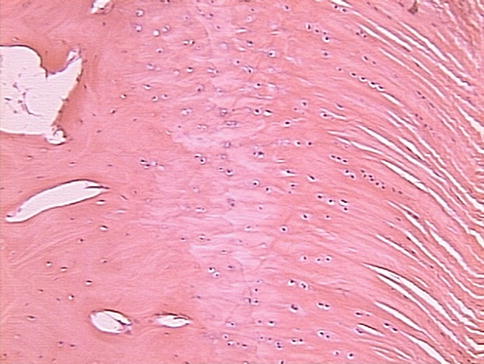
Fig. 21.3
Section of an intact enthesis, showing the four zones (bone, mineralized cartilage, unmineralized cartilage, and tendon) (From Schär and Rodeo [73])
Zone I: This zone constitutes the tendon and is composed of type I collagen fibers that have been well-aligned, and is also contributed by a small percentage of decorin.
Zone II: This zone constitutes fibrocartilage, and it is mainly composed of type II and III collagen, with a small percentage being contributed from types I, IX, and X collagen, as well as some aggrecan and decorin. This zone represents the start of the shift from tendinous to bony tissue.
Zone III: In this zone, mineralized fibrocartilage is predominant. Here, primarily type II collagen, and a high percentage of type X collagen and aggrecan are found. This zone represents the shift toward bony material.
Zone IV: This zone is composed of bone. This zone has a high mineral content and is composed of type I collagen.
In fibrocartilaginous tissue, the main cell population is constituted by chondrocytes that are arranged in rows, which follow the tensile strains of the tendon [31, 32]. The mineral content gradient seems to be one of the main characteristics of the enthesis. It has been shown that mineral clusters and collagens fibrils seem to interdigitate until they reach the fully mineralized region.
21.4.3 Pathology of the Enthesis and Tendon
21.4.3.1 Pathological Changes That May Lead to Rotator Cuff Tear
Rotator cuff tears are one of the most common orthopedic injuries and are the leading cause of shoulder pain and disability. In most cases, a chronic degeneration leads to rupture, although an acute traumatic event may cause tears even in younger patients. The etiology of this degeneration is multifactorial, and can be attributed to both intrinsic and extrinsic factors.
Although detachment could occur in the middle of the tendon or intramuscular zone inside the rotator cuff in traumatic cases, most detachments occur where the mechanical forces change dramatically: the enthesis [33].
It has been suggested by Ogata and Uhthoff that tendon degeneration is the primary cause for partial tears of the rotator cuff [34]. Different pathological changes haven been suggested to lead to degeneration of the rotator cuff tendon, eventually increasing the likelihood of a rotator cuff tear.
Increased Collagen Type III Gene Expression
Neviaser et al. [35] showed in an experimental model that gene expression for Col-III increases in tendons after cyclic fatigue loading. This finding is supported by an altered expression of TGF-β1 (which regulates collagen production in cuff), both in overloaded and torn tendons [36]. Such changes in collagen properties could lead to macroarchitectural changes.
Type I collagen, the most common type of collagen inside rotator cuff tendons, is frequently replaced by type III collagen (usually present in scar tissue) during degeneration. This has less cross-links in between, and consequently worse mechanical properties [37].
Type II collagen, instead, is most frequently seen on the chondral surface of the attachment to bone (zone II and zone III). There is also a shift toward type III collagen during degeneration [38].
These changes could lead to a reduction in strength and a subsequent rupture at the enthesis.
Increased Apoptosis, Oxidative Stress and Autophagy
Tenocytes, in addition to collagen, are of great interest in tendon degeneration. Their number initially decreases, and then a structural change occurs with rounding of the nuclei and apoptosis. This may lead to a change in the structural properties of the tendon, impairing the capability of tenocytes to produce normal healthy collagen. It has also been documented that degenerative tendons have a higher rate of cells undergoing apoptosis compared to histologically normal tendons [39]. Apoptosis may be caused by oxidative stress, with an increase of oxygen-reactive species and JNK (Map-K) expression [40, 41]. Their presence in both torn and unhealthy tendons is not surprising and could lead to a decreased cellular response to damage. As in the muscle belly, it has been observed that autophagic cell death is present as well, suggesting common degenerative mechanisms but with unclear pathways [42] (Table 21.2).
Table 21.2
Cellular characteristics in the extracellular matrix
Cellular characteristics | Extracellular matrix grade | |||
---|---|---|---|---|
0 | 1 | 2 | 3 | |
Autophagic cell death (%) | 3.9 ± 3.6 (51 fields) | 42.9 ± 1.8 (209 fields) | 1.9 ± 1.5 (371 fields) | 46.0 ± 1.8 (269 fields) |
Apoptotic cell death (%) | 21.4 ± 2.2 (54 fields) | 26.0 ± 1.4 (237 fields) | 31.0 ± 1.2 (363 fields) | 34.8 ± 1.6 (246 fields) |
Myofibroblasts (%) | 6.7 ± 1.0 (50 fields) | 13.8 ± 0.9 (229 fields) | 16.9 ± 1.0 (358 fields) | 19.8 ± 1.3 (263 fields) |
Cell density (cells/mm2) | 555 ± 41 (57 fields) | 674 ± 27 (246 fields) | 529 ± 17 (358 fields) | 395 ± 17 (239 fields) |
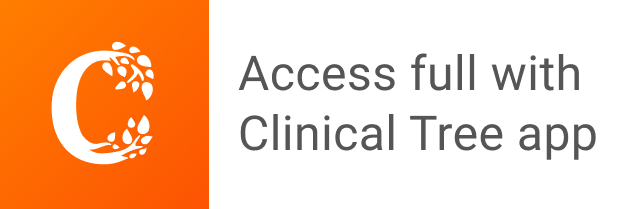