Fig. 1
Images from a patient with dysplasia (a) who underwent arthroscopy and periacetabular osteotomy (b). At the time of arthroscopy, she had full-thickness chondral damage at the acetabular rim (c) and underwent microfracture of the lesion (d) (Reprinted from [5], Copyright © 2011 by American Orthopaedic Society for Sports Medicine, by permission of SAGE Publications)
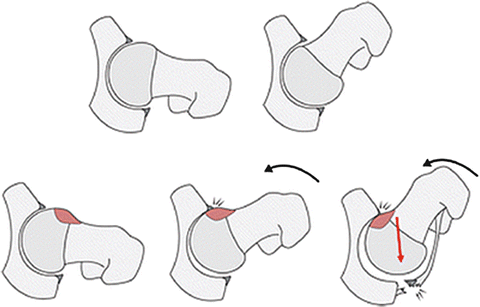
Fig. 2
Episodes of traumatic hip posterior hip dislocation occur with the hip in flexion and internal rotation, with a posteriorly directed force on the femur. If there is a cam lesion present, the femoral head and neck can lever on the anterior acetabular rim. As the femoral head dislocates posteriorly, chondrolabral damage can occur at the posterior labral rim, analogous to a bony Bankart lesion in the shoulder (Reprinted with permission from Springer Science+Business Media, from [6]. Copyright © 2012, The Association of Bone and Joint Surgeons®)
When compared to what is known about cartilage restoration in the knee, there is relatively little information specifically about cartilage restoration in the hip. Currently, most cartilage repair strategies for the hip are based on basic science and techniques that were developed for the knee. In the past decade, however, there has been a dramatic improvement in magnetic resonance imaging (MRI), arthroscopy, and open surgery for younger patients with hip pain. These improvements should also lead to hip-specific cartilage science and restoration techniques. The objective of this chapter is to describe the current state of the art for treating focal articular cartilage defects in the hip. To support this objective, we review the basic structure and function of articular cartilage as well as the biomechanics of the hip and focal articular defects. Subsequently, the clinical presentation, diagnosis, and suspected underlying causes of damage to articular surfaces in the hip are reviewed. Finally, we discuss the available treatment options, their relative indications, and their published outcomes to date.
Cartilage Basic Science: General
Articular cartilage must resist mechanical loading modes that include compression, tension, and shear. The material characteristics of articular cartilage are optimized for these roles and are intricately related to structure and material composition. This section provides a brief review of these topics.
Structure and Composition
Articular cartilage is composed primarily of water, collagen, and large proteoglycans (Fig. 3, left panel). By wet weight, cartilage is 68–85 % water, 10–20 % collagen, 5–10 % proteoglycan, and <5 % other matrix molecules [8]. The interstitial fluid contains dissolved electrolytes, predominantly Na+, Ca2+, Cl−, and K+. Chondrocytes account for less than 10 % of the total tissue volume [9] and are responsible for the metabolic activity of cartilage. The primary collagen in articular cartilage is fibril-forming type II collagen with variable orientation of collagen fibers depending on the depth of the tissue. In the superficial zone (top 10–20 %), fibers are oriented parallel to the articular surface; in the middle zone (middle 40–60 %), fibers are oriented randomly; and in the deep zone (bottom ~30 %), the fibers are oriented perpendicularly to the subchondral bone. Aggrecan accounts for 80–90 % of all proteoglycan in cartilage. Chondroitin sulfate, keratin sulfate, and hyaluronan are the primary glycosaminoglycan (GAG) side chains in cartilage. Chondroitin sulfate and keratin sulfate have negatively charged sulfate and carboxyl groups that make the chains anionic overall. Hyaluronan is not sulfated and interacts with aggrecan and link proteins to form large aggregates that are immobilized in the extracellular matrix and which consequently stabilize the extracellular matrix. Since these charged proteoglycans are immobilized within the extracellular matrix, the resulting charge is referred to as the “fixed charge density.” The proteoglycan distribution, and therefore the distribution of fixed charge density, varies through the cartilage depth. Aggrecan level is the lowest in the superficial zone and increases with depth [8].
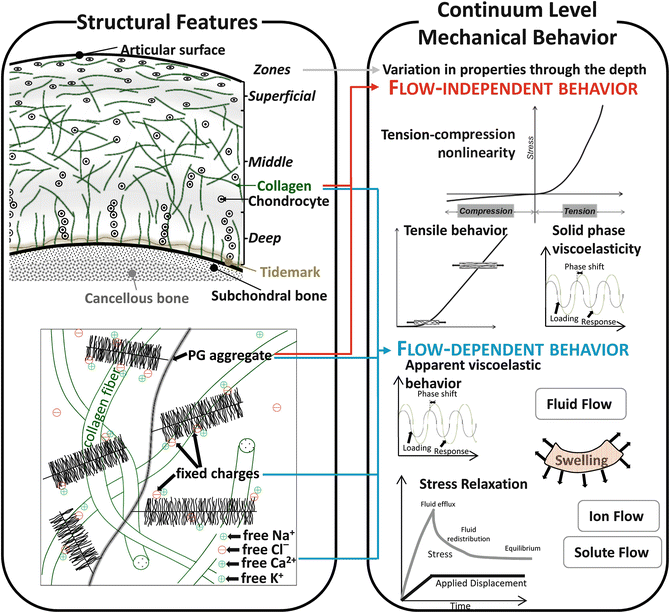
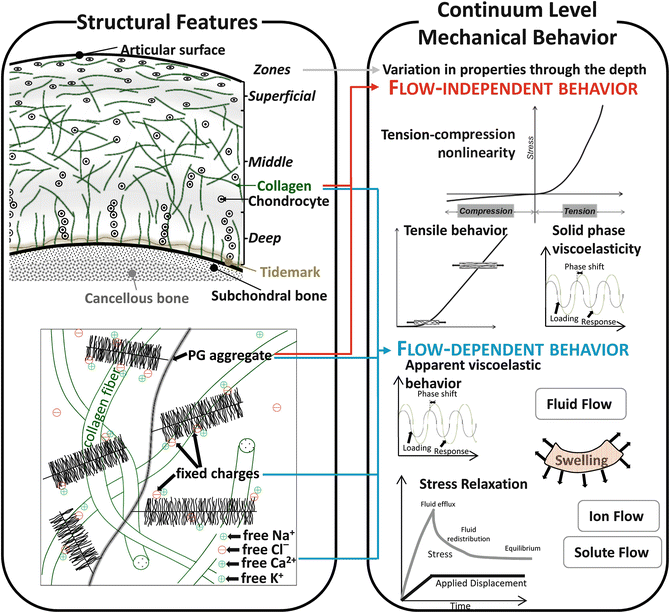
Fig. 3
Cartilage structural features and their relationship to continuum level mechanical behavior. Left panel – the structure and orientation of collagen and proteoglycan aggregates determine the continuum level mechanical behavior. Right panel – key features of continuum mechanical behavior include tension–compression nonlinearity, anisotropy, viscoelastic material behavior, and swelling
Material Properties
The structure of articular cartilage produces complex material behavior (Fig. 3, right panel). Because cartilage structure and composition vary with depth, material properties also vary with depth [10–13]. The material behavior of cartilage varies between species within the same joint, between joints within species, and spatially within each joint within each species [14–16]. Cartilage exhibits nonlinear material behavior in both tension and compression. Under uniaxial tensile stress, cartilage material behavior is primarily determined by the collagen fibrils. The stress–strain curve in tension exhibits a toe region followed by an approximately linear region due to the uncrimping of collagen fibers followed by loading of straightened fibers [8]. Under uniaxial compressive stress, the material response of cartilage is governed by the proteoglycan matrix and fluid flow. The modulus of cartilage in tension is approximately one to two orders of magnitude lower than in compression, a discrepancy known as tension–compression nonlinearity [16]. This characteristic is important for most modes of cartilage deformation that are relevant to whole-joint mechanics.
Fluid–solid interactions, the intrinsic viscoelasticity of the solid phase, and fixed charge density causes flow-dependent viscoelasticity of the cartilage and swelling [17–19]. The cartilage swelling is caused by the fixed charge density of the tissue and charge–charge repulsion between closely packed GAGs attracting interstitial fluid counterions [8]. Collagen primarily bears tensile stress, and thus the fibrillar collagen resists expansion of the solid matrix during swelling [20].
Solutes, including nutrients and metabolic by-products, move through cartilage via diffusion. Solute diffusivity in cartilage is smaller than in aqueous solution [8], and diffusivity decreases as the tissue is compressed [21, 22]. The size of the solute also influences solute diffusivity. For large solutes, cyclic loading can enhance diffusion but it has no effect on small solutes [23].
Mechanics of the Hip
The kinematics (motion) of the hip are primarily determined by the congruency of the articular surfaces, their shape and curvature, the limits of motion imposed by bony contact between the femur and acetabulum or labrum, and the joint reaction forces related to musculature and body weight. The stress at the articular cartilage layers and the cartilage contact area during articulation are determined by joint congruency, curvature, chondral thickness and material properties, and joint load. Local joint congruency, defined as the congruency of the two articulating surfaces in the region of a particular point of contact, is in turn a function of joint kinematics.
The magnitude and orientation of the abductor muscle force as well as the distance from the joint center counteracts the force from body weight, so that the overall joint reaction forces across the hip are determined by the sum of these two oppositely directed forces. Typical joint reaction forces during single-leg stance are approximately three times the body weight but vary as a function of activity. The study by Bergman et al. is the most widely quoted report on in vivo hip joint reaction forces [24]. These investigators measured hip joint reaction forces during activities of daily living in patients who had received a total hip replacement with a telemeterized load cell. Joint reaction forces ranged from 2.4 times body weight during level walking to 2.6 times body weight when walking downstairs. Independent of other factors, joint reaction forces are decreased by medializing the center of rotation. For example, this can occur nonsurgically by the use of a cane in the contralateral hand, or surgically via lateralization of greater trochanter, which moves the relative point of application of the abductor muscles farther from the joint center.
Although the normal hip is generally considered to be a highly congruent joint, the local congruency varies with changes in joint orientation, such as flexion–extension or abduction–adduction. Further, congruency is often altered in joints with pathomorphologies such as acetabular dysplasia and FAI. For a given joint reaction force, higher congruency generally implies a larger contact area and thus lower contact stresses. If the global congruency is defined as, for instance, the congruency of a pair of spheres to the articular surfaces, dysplastic hips have a lower global level of congruency than normal hips. However, this does not necessarily translate into higher local contact stresses since local congruency is not significantly different between normal and dysplastic hips [25].
Contact Patterns
Areas of contact on the articular surfaces vary somewhat with activity and motion during the activity in both normal and pathomorphologic hips. In the normal hips, the direction of loading and the location of chondral contact change from predominantly superior–posterior during ascending stairs, to more superior during walking, to superior–anterior during descending stairs [26]. Predicted peak stress in normal hips ranges from 7.52 ± 2.11 MPa for heel-strike during walking (2.3 times the body weight) to 8.66 ± 3.01 MPa for heel-strike during descending stairs (2.6 times the body weight). Across all activities of daily living, the contact area of the femur on the acetabular cartilage occupies about a third of the total surface area. Even in the normal hips, the distribution of contact stress is highly nonuniform due to local incongruities between the femoral and acetabular cartilage. In addition, contact stress varies more between different subjects for a single activity than between different activities for a single subject.
In the hips with acetabular dysplasia, the acetabulum is shallow. Dysplasia is typically diagnosed radiographically by an anterior and/or lateral center edge angle (CEA) less than 20–25° and an acetabular index greater than 10°. These measures are indicative of a shallow acetabulum and an upwardly sloping sourcil, respectively. Patient-specific finite element (FE) predictions of contact area show that only the superior region of the acetabulum exhibits significantly different labral contact areas when comparing normal and dysplastic subjects [25] (Fig. 4). This suggests that during activities of daily living, the superior labrum in the dysplastic hip is loaded more than other portions of the acetabular labrum. Predictions of labral load support corroborate this finding – the labrum in dysplastic hips supports loads that are 2.8–4.0 times larger than that of normal hips. These results are consistent with clinical observations of labral hypertrophy, as well as the superior or anterosuperior location of labral tears in the dysplastic hip [27–29].
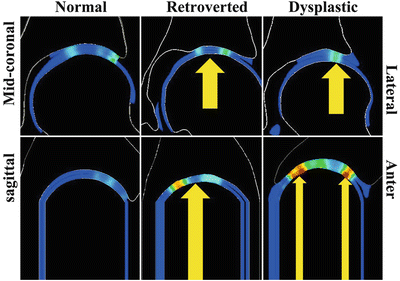
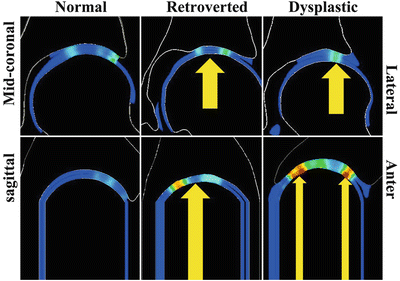
Fig. 4
Cartilage contact pressure in coronal (top) and sagittal (bottom) slices of a representative normal hip (left), retroverted hip (middle), and dysplastic hip (right). Normal hips exhibited centered, distributed loading (left column). Contact in retroverted hips was moved medially and superiorly with respect to normal hips (middle column). In dysplastic hips, loading was shifted laterally, and in comparison to normal hips, a significantly higher percentage of load was supported by the acetabular labrum [25, 30]
In the hips with acetabular retroversion, the acetabulum opens more posterolaterally than normal. This is recognized on anteroposterior radiographs by the presence of a crossover sign, which indicates a prominent anterior acetabular wall, a deficient posterior acetabular wall, or both. Cartilage contact in retroverted hips is focused in the superomedial acetabulum, whereas in normal hips, contact patterns are distributed over more of the entire acetabulum (Fig. 4) [30]. Additionally, retroverted subjects tend to have patches of more medial contact.
Mechanical Causes of Articular Cartilage Defects
Focal cartilage defects often result from cartilage delamination, typically due to shear loading that causes failure at the osteochondral interface. In vitro, both articular surface contact stress and maximum shear stress have been shown to predict cartilage fissuring under impact loads [31, 32]. The maximum shear stress during activity for the normal hip tends to occur at the interface of the acetabular cartilage and subchondral bone, near the junction of the cartilage with the labrum [33].
For a specific joint, chondral defects greater than a certain size tend to increase in size, generate an inflammatory response in the joint, and ultimately lead to OA, while other smaller defects will remain stable and not progress to disease [34]. Furthermore, a number of geometric factors contribute to the variability in clinical success treating osteochondral defects , as these factors often produce unfavorable conditions for the formation of new cartilage or the survival of implanted plugs, scaffolds, or cells [35]. The major geometric factors are defect size, joint curvature, joint congruence, cartilage thickness, and status of the rim of the defect. Increased joint curvature generally causes the rim of the defect to experience higher stresses and strains during joint loading and articulation. This is further exacerbated if the joint surfaces are incongruent. Thinner regions of articular cartilage have less ability to increase the local congruency during deformation since deformation is limited by the reduced thickness. And, finally, if the rim of the defect is well defined and intact, the defect will be more likely to remain stable and not progress in the joint, whether it is repaired or not.
As illustrated in the upper panels of Fig. 5 for a defect in the femoral condyle of the knee, increasing defect size exposes the rim of the defect to higher stresses and strain. Furthermore, in larger defects, healing neocartilage or implanted plugs or cells are more exposed to deformation during healing, resulting in a progressively worse outcome independent of other factors. Because the congruency of diarthrodial joints can vary as a function of joint kinematics, a defect may be subjected to different degrees of loading as a function of joint articulation. This is illustrated in the lower panels of Fig. 5 for the femoral condyle of the knee as a function of knee flexion.
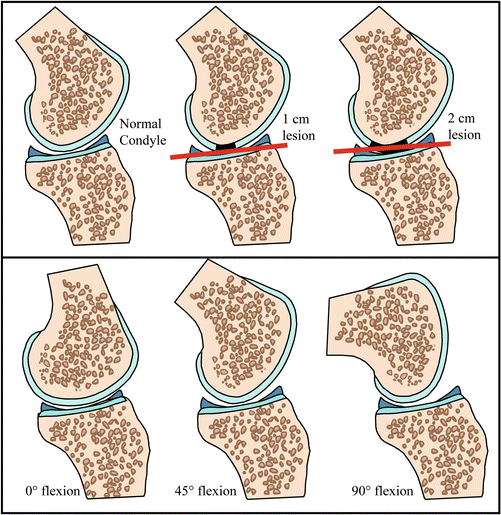
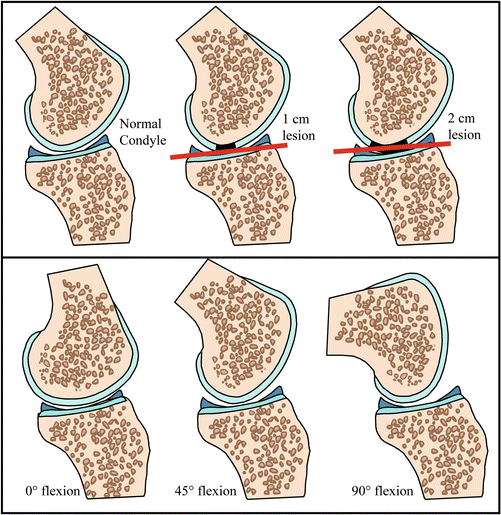
Fig. 5
Effects of defect size and joint curvature on focal cartilage defects, shown for the femoral condyle of the knee joint. Top panels – as defect size increases, the rim of the defect and healing tissue within the defect is subjected to increasingly high strains during normal joint loading. Bottom panels – joint congruency and curvature often vary as a function of joint orientation. These changes have implications for the effective strains that a defect will experience. As knee flexion increases, congruency of the articular surfaces and, thus contact area, decreases while contact stresses and strains increase
Clinical Characteristics of Hip Cartilage Defects
There is no single history or physical examination finding that is pathognomonic for cartilage damage or chondral defects in the hip. There are, however, some predictable patterns of injury. Byrd reported a series of athletes who had chondral damage after lateral impact events, sustaining a blow to the greater trochanter during a fall [7]. Because younger patients typically have little soft tissue to absorb the force of an impact, the force is transmitted to the central joint surface. The cartilage subsequently fails at the medial aspect of the femoral head. There may also be a corresponding lesion in the weight-bearing portion of the acetabulum just above the fossa, which may result from chondrocyte compression [7]. Parafoveal chondral defects have been described in athletes with FAI that participate in sports that require rapid lower extremity flexion, torsion, and force. The lesion seems to occur from impingement-induced instability and translation, with incongruent hip motion causing high shear stresses and cartilage deformation [4]. Similarly, athletes who have a distinct hip subluxation or dislocation can have chondral injuries and ligamentum teres tears around the fovea, as well as posterior labrum and cartilage injury [6]. Finally, about 25 % of patients with a history of Perthes’ disease in childhood have full-thickness chondral defects [3]. Regardless of the proposed etiology, patients report some combination of sharp groin or buttock pain, stiffness, clicking, popping, or catching [7, 36]. These symptoms are also characteristic of labral tears, and patients with chondral defects frequently have a coexisting labral tear [36].
No single exam maneuver is specific for chondral pathology. The location of the lesion may influence the presence and nature of pain with weight-bearing or with specific exam maneuvers. Pain with hip “logrolling” usually indicates synovitis and/or a hip joint effusion. A full hip examination including stance, gait, range of motion, strength, location of specific sites of tenderness, and provocative maneuvers including apprehension and impingement testing should be performed. It is important to evaluate for FAI or dysplasia, labral tears, compensatory tendinopathies, and compensatory gait patterns. Both FAI and dysplasia can cause or exacerbate chondral damage and should be addressed if surgery is planned.
All patients should have radiographs of the hip to evaluate for FAI, dysplasia, joint incongruity, and early arthritic changes. A CT scan with version analysis is helpful for further evaluation of FAI or dysplasia, but does not image the cartilage directly. Cartilage defects are best seen with a high quality MRI or MR arthrogram of the hip. The MRI should be performed with a 1.5T or 3T magnet and small-field-of-view coil. A combination of coronal, sagittal, axial, and radial images best characterizes the location, size, and nature of the defect. Cartilage imaging is, however, notoriously difficult. MRA is more effective for detecting labral tears than cartilage damage, with sensitivity for chondral defects ranging from 47 % to 81 %, specificity ranging from 66 % to 89 %, and accuracy of 67 % [36, 37]. Bony edema adjacent to the defect can indicate recent trauma [7], or local overload [36].
Treatment Options
The treatment goals for cartilage restoration surgery are the resolution of symptoms, return to activity, and prevention of progressive damage. To achieve this, a preoperative plan is essential, and the nature of the lesion including size, location, underlying etiology, or associated structural pathoanatomy must be known. Indications for treating a focal chondral defect in the hip include acute trauma with an unstable fragment, continued pain and symptoms despite conservative management, a visible chondral defect on preoperative imaging with a positive response to a diagnostic intra-articular injection, and intra-articular loose bodies. The timing of surgery depends on the type of lesion, age of the patient, and any previous interventions. Of note, patients who have loose bodies or unstable fragments should undergo surgery in a more urgent fashion because of the potential for severe cartilage damage from a loose fragment within the joint.
Treatment options for cartilage defects include conservative measures like activity modification, weight management, and viscosupplementation as well as surgical techniques like microfracture, second-generation ACI techniques, and osteochondral allografts (Figs. 6, 7, and 8) [38, 39]. Specific technique chapters follow for each type of cartilage restoration surgery; the discussion of each type of intervention in this chapter will focus on the basic science rationale for each technique and any published outcomes for the hip.
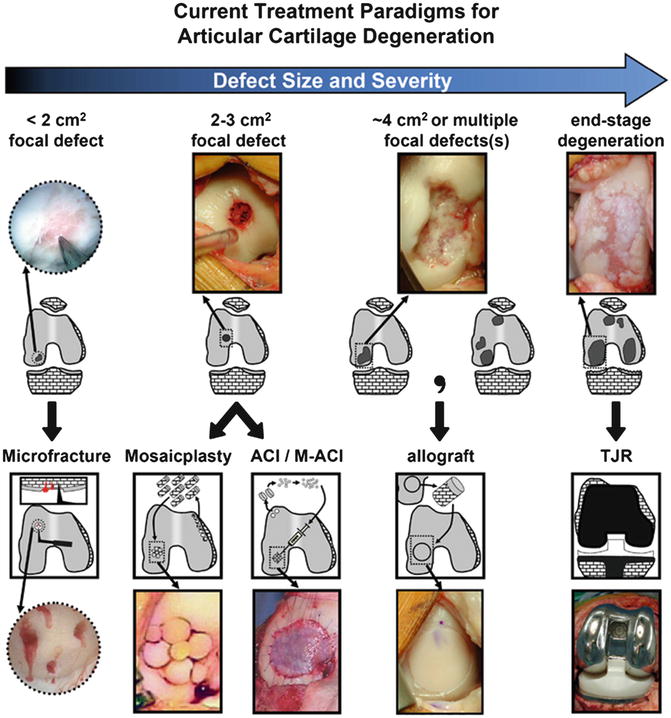
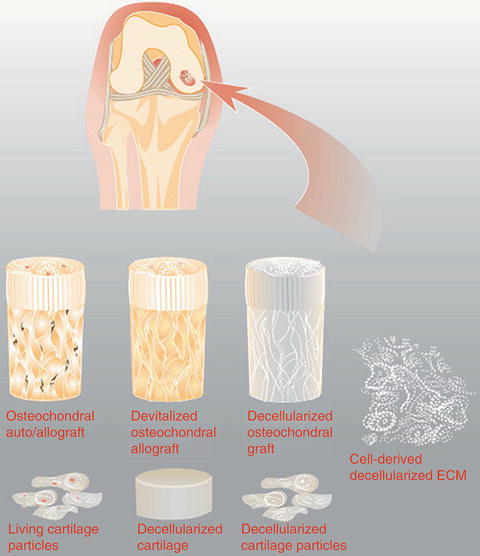
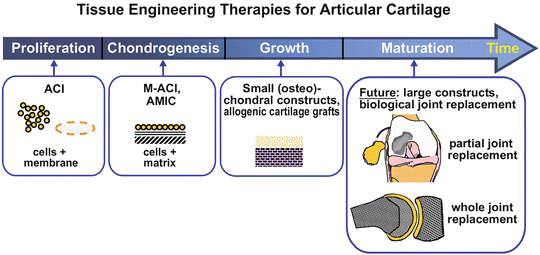
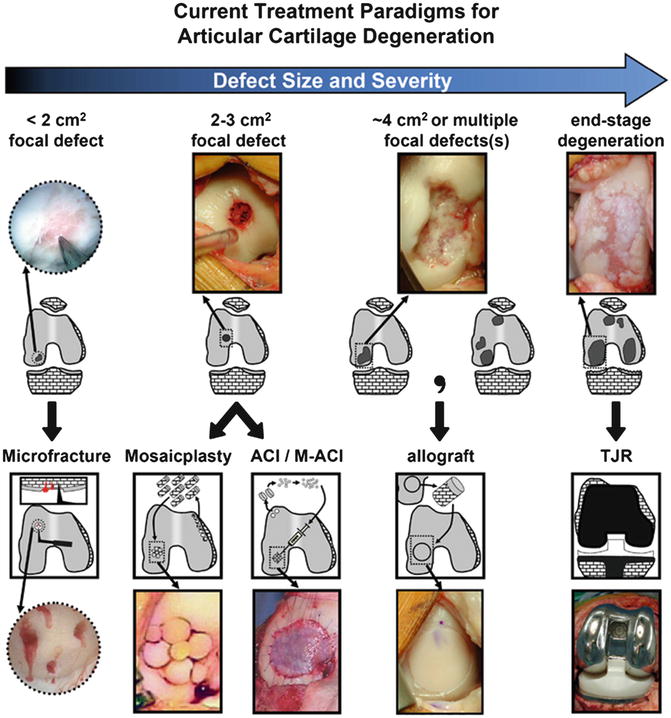
Fig. 6
Currently, treatment decisions for cartilage restoration are based on the size and type of lesion (here, depicted for the knee). Small focal defects (<2 cm2) are often treated with microfracture. Larger defects (2–3 cm2) can be treated with osteochondral autograft or cell-based therapies like ACI, MACI, AMIC, or allogenic chondrocytes. Even larger defects (~4 cm2) are treated with allografts (Reprinted from [39] with permission from Elsevier)
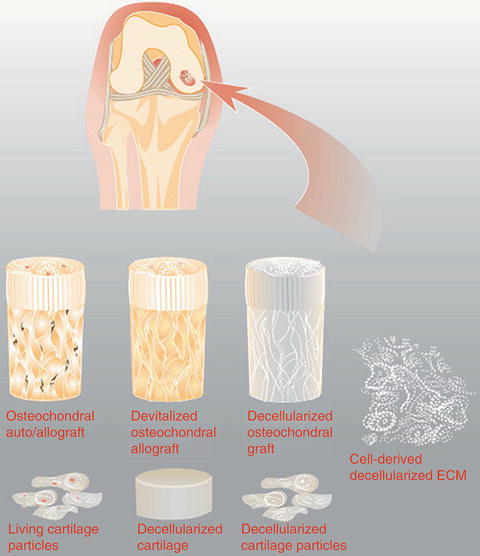
Fig. 7
The treatment strategy for a chondral defect can also be considered in the context of the matrix and scaffold being applied. Currently used grafts may consist of cells and matrix, as in the case of an osteochondral allografts and autografts, and juvenile cartilage particles, or just matrix as is the case with devitalized grafts. In the future, cell-derived decellularized ECM produced in vitro may also be a treatment option (Reprinted from [38] with permission from Elsevier)
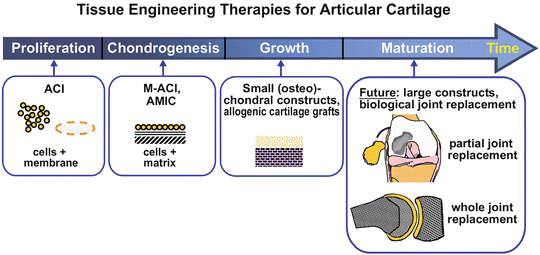
Fig. 8
Developmental progression of tissue-engineering therapies for articular cartilage repair. In general, cartilage repair therapies are based on a strategy of reproducing normal cartilage growth and development. Early strategies like ACI rely on chondrocyte proliferation. Second-generation therapies like MACI or AMIC involve cells implanted in a matrix and covered with a membrane with the goal of producing in situ cartilage. More recently developed therapies include implantation of juvenile cartilage, with the goal of expansion and development into mature adult cartilage tissue. Future directions include development of larger constructs and potentially a biological joint replacement (Reprinted from [39] with permission from Elsevier)
Nonoperative Management
For many patients with chondral defects, a trial of nonoperative measurement is appropriate. This often consists of activity modification or weight management to decrease the mechanical load across the damaged area of cartilage. Physical therapy focusing on hip and core strengthening may also help improve the muscular dynamics around the hip, particularly if there is a component of instability [40, 41]. Patients frequently ask about nutritional supplements. The most well known of these is a combination of glucosamine and chondroitin. Individual trials have shown mixed results, and in meta-analysis, there was no clinically relevant effect on joint pain or joint space narrowing [42].
Intra-articular cortisone injections may be used to reduce symptoms from inflammation. However, each injection has some risk of infection, soft tissue weakening, and possibly a microscopic effect on the cartilage [43]. Multiple cortisone injections should not be the definitive management strategy for young patients who are potential candidates for cartilage restoration procedures. Platelet-rich plasma (PRP) has also been touted as a possible treatment for cartilage defects or osteoarthritis. Advocates for intra-articular PRP injections promote it as a topical application of biological factors that promote healing of the defect. However, preparations of PRP vary and although the effective agent has been proposed, it is not definitively known [44]. There are two studies of PRP injection for hip arthritis; other studies of intra-articular PRP are for the knee or for the talus. Most patients have some initial improvement with gradual worsening over time [45]. Hyaluronic acid or viscosupplementation improves symptoms by a combination of antiinflammatory effects, restoration of the viscosity of synovial fluid, and normalization of synovial cell hyaluronate synthesis [46]. It appears to be safe, without potential adverse effects [46]. The results of hyaluronic acid injections are mixed. In the hip, 45–60 % of people have pain relief 6 months after injection [46].
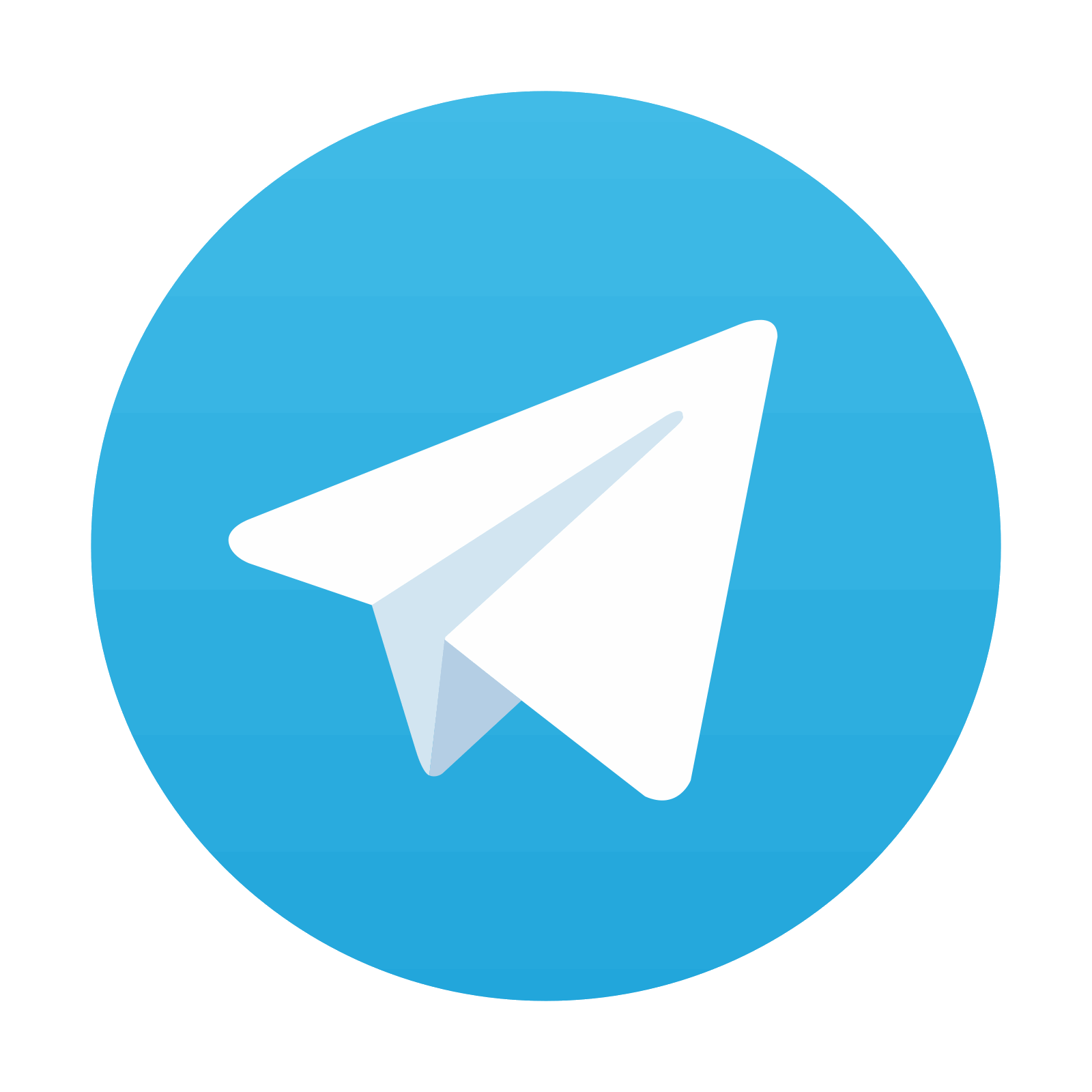
Stay updated, free articles. Join our Telegram channel
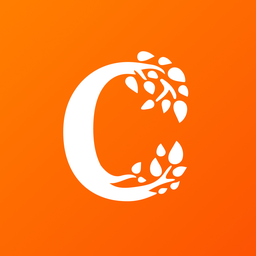
Full access? Get Clinical Tree
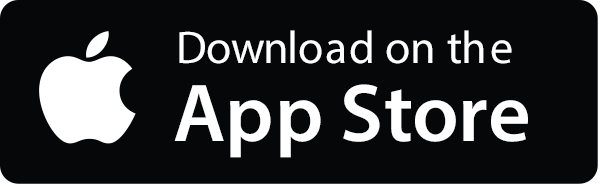
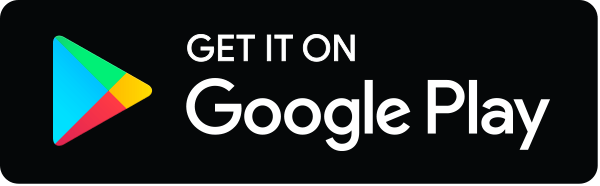