Clinical Spectroscopy
Thomas H. Berquist
Magnetic resonance spectroscopy (MRS) was developed prior to magnetic resonance imaging (MRI).1,2 MRI provides excellent anatomic detail and clearly defines pathology due to its superior contrast and flexibility of image plane selection compared with computed tomography (CT) and other imaging techniques. MRS offers a noninvasive technique for evaluating biochemical changes in situ.3,4 Although MRS has progressed as a clinical tool, even at the time of this sixth edition, it is still not universally used clinically.1,4,5,6 Bottomley4 described four challenges for MRS that needed to be accomplished before the technique becomes an accepted clinical tool. These challenges include providing technical feasibility in a clinical setting, interpreting and understanding the new chemical data in clinical medicine, obtaining adequate data on normal and pathologic tissues, and developing MRS into an efficacious diagnostic technique.4
MRS continues to be an effective research tool, especially with new 3- to 7-T systems.3,4,5,6,7 In recent years, more applications have evolved using hydrogen (1H), phosphorus (31P), sodium (23Na), carbon (13C), and choline to study neurologic, cardiac, liver, breast, prostate, myopathies and bone, and soft tissue neoplasms.3,8,9,10,11,12,13,14,15,16 New techniques and higher field strength whole body imagers (3 to 8 T) have contributed to the expanded utility of MRS.3,8,17,18,19
This chapter provides review of current clinical applications and future potential. Certain basic principles are reviewed primarily as they relate to clinical practice. An in-depth discussion of the physics of MRS is beyond the scope of this chapter.
BASIC PRINCIPLES OF MAGNETIC RESONANCE SPECTROSCOPY
Clinical MRS research has concentrated on 31P, 1H, 13C, 23Na, fluorine (19F), and, most recently, choline.1,3,8,20-23 1H and, to a lesser degree, 31P have been most commonly studied because of the need to obtain adequate signal to noise ratio (SNR) and clinically significant data.2,3,24,25 Although potentially useful, 13C spectroscopy has also lagged behind due to significant modifications required for conventional clinical imaging systems.25
Because of the improved spectra from smaller tissue volumes at clinical field strengths (1.5 T), much of the recent clinical research has shifted from phosphorus to hydrogen spectroscopy.3,25,26,27 New approaches may evolve with the increased use of higher (3 to 8 T) field strength magnets. However, to date, there have been problems at higher field strength due to issues with shimming, T2 contraction, field inhomogeneity, and magnetic susceptibility effects.3,4,28
When a given tissue sample is studied, the results depend upon the magnetic field strength, temperature, metabolic concentration, and sample size. Sensitivity and localization of the sample are critical. Even when coil selection is optimal, overlying tissue can reduce the signal.2
To date, there is no consensus as to which localization technique is best. Six techniques have been commonly used2,20,29: rotating frame zeugmatography, topical MR, depth-resolved surface coil spectroscopy, image-selected in vivo spectroscopy, one- to three-dimensional phase encoding or spectroscopic techniques, and chemical shift imaging. This group of techniques fall into three categories of spatial localization: spatial gradients in a radiofrequency field (i.e., rotating frame zeugmatography), static spatial gradients in the main magnetic field (i.e., topical MR), and pulsed spatial gradients at audio frequencies (i.e., depth-resolved surface coil spectroscopy, image-selected in vivo spectroscopy).4
In recent years, new techniques have been developed to improve clinical MRS. These include magnet designs, coils, sequences, and filters.9,11,26,28,30,31 New coil designs have significantly improved spatial resolution.32,33,34 Truncation effects for K-space sampling with two-dimensional MRS can be improved by using filters to improve image representation of the tissue being evaluated.19 New fast pulse sequences such as rapid acquisition with relaxation enhancement (RARE) have improved SNRs.17 MRS can also be enhanced when combined with diffusion weighted imaging, diffusion tensor imaging, or dynamic contrast studies.26
Also, from a practical standpoint, one should consider whether contrast enhancement, which is used much more commonly today, affects MRS data. Studies suggest that there is no effect on MRS data.18
Most clinical and research studies with in vivo spectroscopy have focused on 1H, 31P, and, to a lesser degree, 13C.1,4,12,20,21,26,27,28,29,33,34
31P is most often studied for its fundamental importance in energy metabolism and membrane construction
(Fig. 16.1).1,3,35,36 Signal intensity is 1,000,000 times less than 1H, so achieving adequate SNR can be difficult.1 Field strengths of 1.5 T or greater are required.
(Fig. 16.1).1,3,35,36 Signal intensity is 1,000,000 times less than 1H, so achieving adequate SNR can be difficult.1 Field strengths of 1.5 T or greater are required.
Glycolysis → ATP and lactic acid production.29
1H spectroscopy is more often used than 31P spectroscopy due to abundance, higher SNR, and performance at conventional MR field strengths of 1.5 to 3 T.3,4,27,34 Hydrogen spectroscopy provides a profile of mobile metabolites in human tissue including N-acetyl aspartate, creatinine, glutamate/glutamine, and choline, in addition to lactate noted earlier.26 Hydrogen spectroscopy clearly demonstrates two large peaks (Fig. 16.2); a water peak and CH2
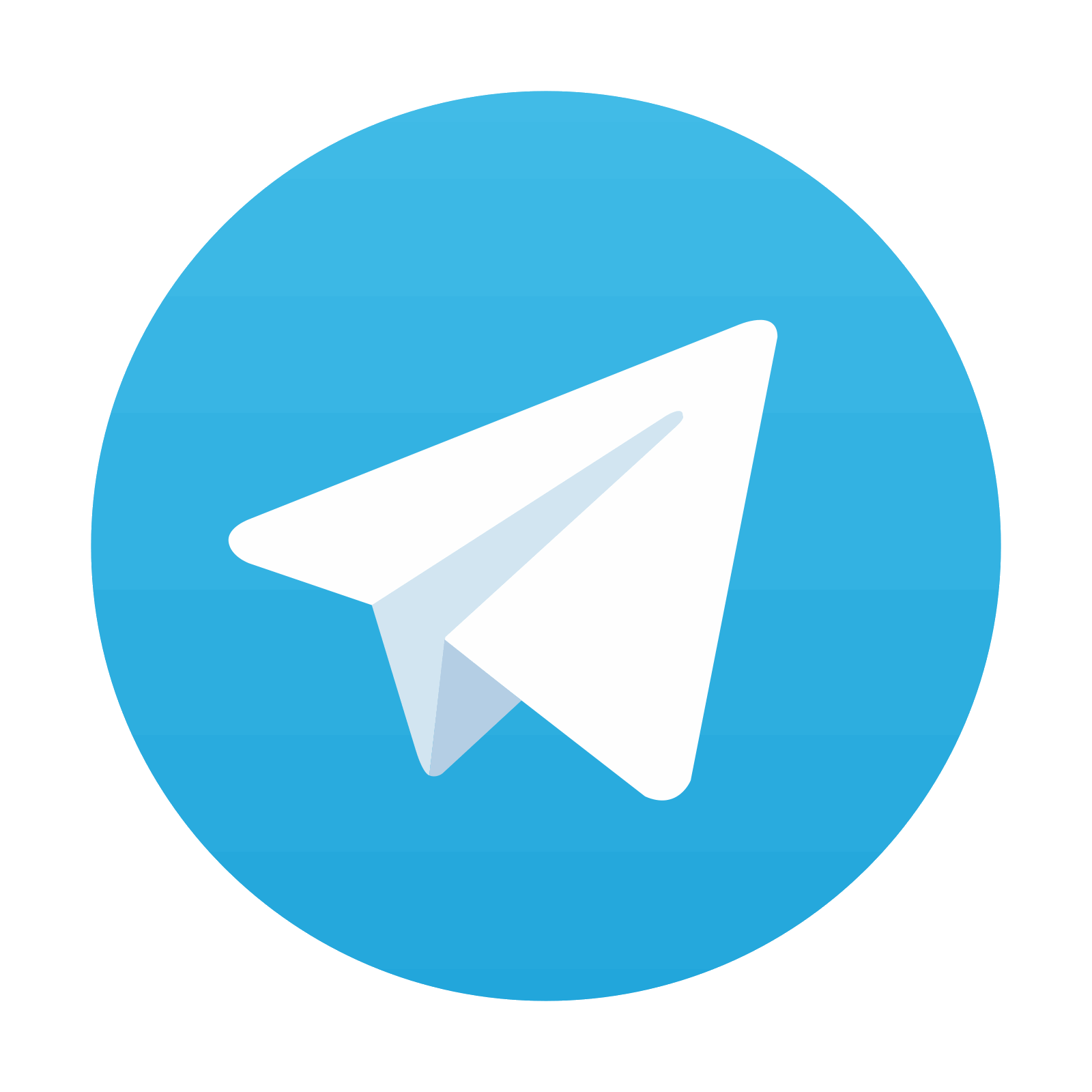
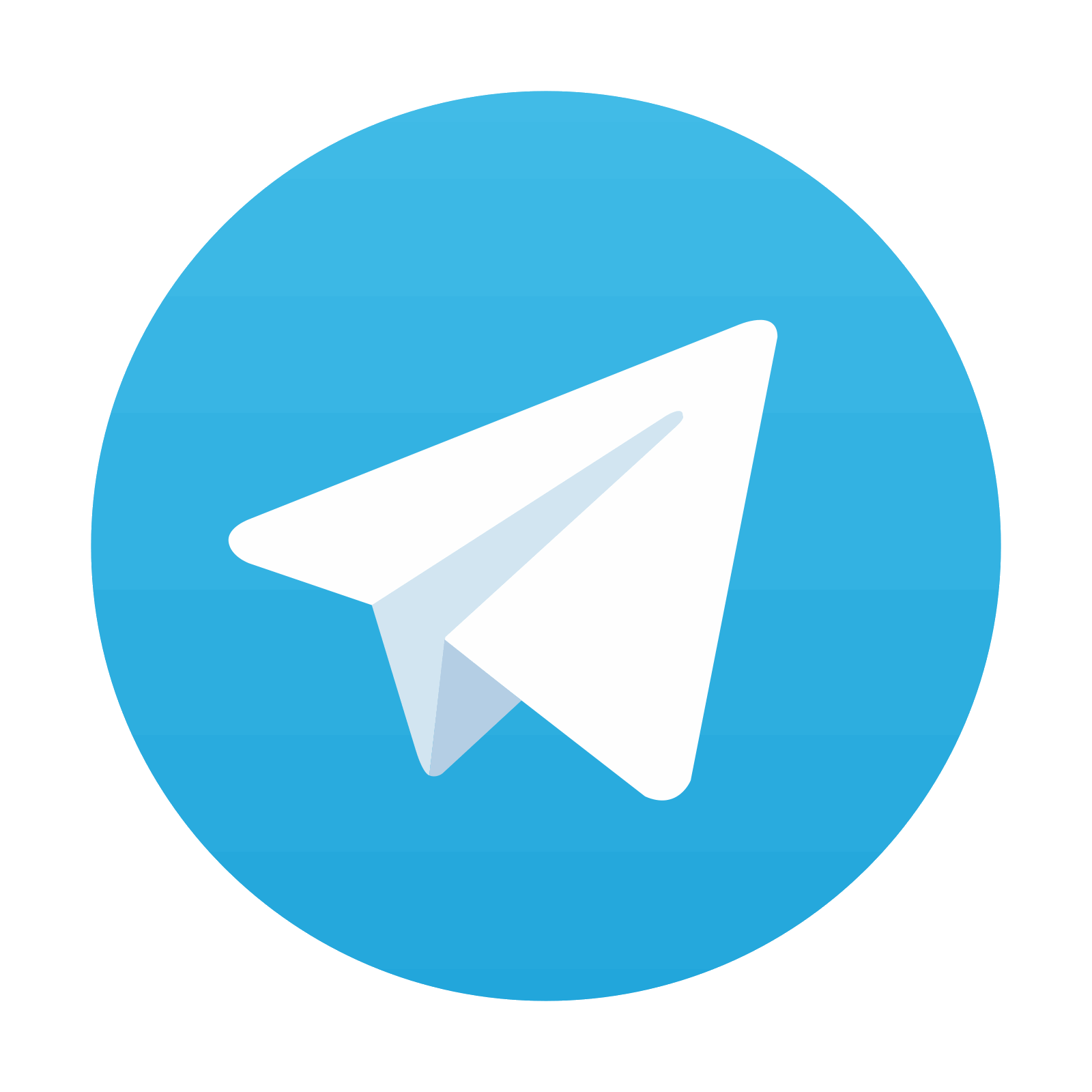
Stay updated, free articles. Join our Telegram channel
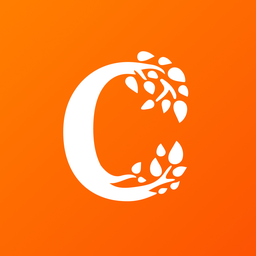
Full access? Get Clinical Tree
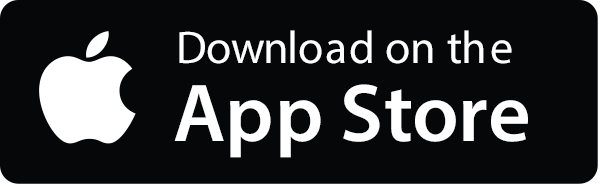
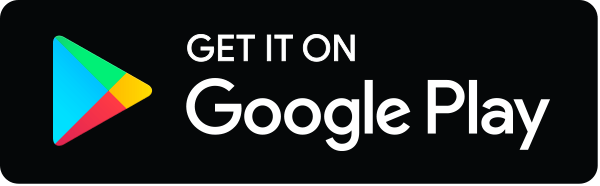