1 Biology of the Normal Joint
Classification of Joints
Human joints provide the structures by which bones join with one another and may be classified according to histologic features of the union and range of joint motion. Three classes of joint design have been identified: (1) synovial or diarthrodial joints (Figure 1-1), which articulate with free movement, have a synovial membrane lining the joint cavity, and contain synovial fluid; (2) amphiarthroses, in which adjacent bones are separated by articular cartilage or a fibrocartilage disk and are bound by firm ligaments permitting limited motion (e.g., pubic symphysis, intervertebral disks of vertebral bodies, distal tibiofibular articulation, sacroiliac joint articulation with pelvic bones); and (3) synarthroses, which are found only in the skull (suture lines), where thin, fibrous tissue separates adjoining cranial plates that interlock to prevent detectable motion before the end of normal growth, yet permit growth in childhood and adolescence.1
Developmental Biology of the Diarthrodial Joint
Skeletal development is initiated by the differentiation of mesenchymal cells that arise from three sources: (1) neural crest cells of the neural ectoderm that gives rise to craniofacial bones; (2) the sclerotome of the paraxial mesoderm, or somite compartment, which forms the axial skeleton; and (3) the somatopleure of the lateral plate mesoderm, which yields the skeleton of the limbs.2 The appendicular skeleton develops in the human embryo from limb buds, which first are visible at around 4 weeks of gestation. Structures resembling adult joints are generated at approximately 4 to 7 weeks of gestation.3 Many other crucial phases of musculoskeletal development follow, including vascularization of epiphyseal cartilage (8 to 12 weeks), appearance of villous folds in synovium (10 to 12 weeks), evolution of bursae (3 to 4 months), and appearance of periarticular fat pads (4 to 5 months).
The upper limbs develop approximately 24 hours earlier than analogous portions of the lower limbs. Proximal structures, such as the glenohumeral joint, develop before more distal ones, such as the wrist and hand. As a consequence, insults to embryonic development during limb formation affect a more distal portion of the upper limb than of the lower limb. Long bones are formed as a result of replacement of the cartilage template by endochondral ossification. The stages of limb development are well described by O’Rahilly and Gardner3,4 and are shown in Figure 1-2. The developmental sequence of events occurring during synovial joint formation and some of the regulatory factors and extracellular matrix components involved are summarized in Figures 1-3 and 1-4.
Interzone Formation and Joint Cavitation
The morphology of the developing synovial joint and the process of joint cavitation have been described in many classic studies done on the limbs of mammalian and avian embryos.5 In the human embryo, cartilage condensations, or chondrifications, can be detected at stage 17, when the embryo is small, approximately 11.7 mm long.3,4 In the region of the future joint, following formation of the homogeneous chondrogenic interzone at 6 weeks (stages 18 and 19), a three-layered interzone is formed at approximately 7 weeks (stage 21), which consists of two chondrogenic, perichondrium-like layers that cover the opposing surfaces of the cartilage anlagen and are separated by a narrow band of densely packed cellular blastema that remains and forms the interzone. Cavitation begins in the central interzone at about 8 weeks (stage 23).
Although these cellular events associated with joint formation have been recognized for many years, only recently have the genes regulating these processes been elucidated. These genes include growth differentiation factor (GDF)-5, Wnt-14, bone morphogenetic protein (BMP)-2, BMP-4, BMP-6, BMP-7, and the GDF-BMP antagonists.5–8 In addition, joint formation is accompanied by the expression of several fibroblast growth factor (FGF) family members, including FGF-2 and FGF-4.9 The balance of signaling between BMP and FGF determines the rate of proliferation, adjusting the pace of differentiation.10 Two transcription factors, Cux-1, a homeobox factor, and the ETS factor ERG/C-1-1, are expressed concurrently with GDF-5 and Wnt-14 at the onset of joint formation.11,12 Hartmann and Tabin13 have proposed two major roles for Wnt-14. First, it acts at the onset of joint formation as a negative regulator of chondrogenesis. Second, it facilitates interzone formation and cavitation by inducing expression of GDF-5 (also known as cartilage-derived morphogenetic protein-1 [CDMP-1]), Wnt-4, chordin, and the hyaluronan receptor, CD44.13–15 Paradoxically, application of GDF-5 to developing joints in mouse embryo limbs in organ culture causes joint fusion,16 suggesting that temporospatial interactions among distinct cell populations are important for the correct response. The current view is that GDF-5 is required at early stages of condensation, where it stimulates recruitment and differentiation of chondrogenic cells, and later, when its expression is restricted to the interzone.
The distribution of collagen types and keratan sulfate in developing avian and rodent joints has been characterized by immunohistochemistry.17–21 Collagen types I and III characterize the matrix produced by mesenchymal cells, which switch to the production of types II, IX, and XI collagens that typify the cartilaginous matrix at the time of condensation.22 The messenger RNAs (mRNAs) encoding the small proteoglycans, biglycan and decorin, may be expressed at this time, but the proteins do not appear until after cavitation in the regions destined to become articular cartilage.23 Interzone regions are marked by the expression of type IIA collagen by chondrocyte progenitors in the perichondrial layers, type IIB and XI collagens by overt chondrocytes in the cartilage anlagen, and type I collagen in the interzone and in the developing capsule and perichondrium (Figure 1-5).24
The interzone region contains cells in two outer layers that are destined to differentiate into chondrocytes and become incorporated into the epiphyses, and in a thin intermediate zone that are programmed to undergo joint cavitation and may remain as articular chondrocytes.25 Fluid and macromolecules accumulate in this space, creating a nascent synovial cavity. Blood vessels appear in the surrounding capsulosynovial blastemal mesenchyme before separation of adjacent articulating surfaces.26 Although it was first assumed that these interzone cells should undergo necrosis or programmed cell death (apoptosis),27 some investigators have found no evidence of DNA fragmentation preceding cavitation.24,25,28,29 Evidence that metalloproteinases are involved in loss of tissue strength in the region undergoing cavitation is also lacking.30 Instead, the actual joint cavity seems to be formed by mechanospatial changes induced by the synthesis of hyaluronan via uridine diphosphoglucose dehydrogenase (UDPGD) and hyaluronan synthase. The interaction of hyaluronan with its cell surface receptor, CD44, modulates cell migration, but it is thought that the accumulation of hyaluronan and the associated mechanical influences play a major role in forcing the cells apart and inducing rupture of the intervening extracellular matrix by tensile forces.20,30 This mechanism accounts for the observation that joint cavitation is incomplete in the absence of movement.31,32 Equivalent data from human embryonic joints are difficult to obtain. In all large joints in humans, complete joint cavities are apparent at the beginning of the fetal period.
Cartilage Formation and Endochondral Ossification
Condensation and Limb Bud Formation
Formation of cartilage anlagen occurs in four stages: (1) cell migration, (2) aggregation regulated by mesenchymal-epithelial cell interactions, (3) condensation, and (4) overt chondrocyte differentiation, or chondrification.3,4,33 Interactions with the epithelium determine mesenchymal cell recruitment and migration, proliferation, and condensation.3,4,34 The aggregation of chondroprogenitor mesenchymal cells into precartilage condensations was first described by Fell33 and depends on signals initiated by cell-cell and cell-matrix interactions, the formation of gap junctions, and changes in the cytoskeletal architecture. Before condensation, the prechondrocytic mesenchymal cells produce extracellular matrix that is rich in hyaluronan and type I collagen and type IIA collagen, which contains the exon-2–encoded aminopropeptide found in noncartilage collagens.35 The initiation of condensation is associated with increased hyaluronidase activity and the appearance of cell adhesion molecules, neural cadherin (N-cadherin), and the neural cell adhesion molecule (N-CAM), all of which facilitate cell-cell interactions.
Before chondrocyte differentiation, cell-matrix interactions are facilitated by fibronectin binding to syndecan, downregulating N-CAM and setting condensation boundaries. Increased cell proliferation and extracellular matrix remodeling, with the disappearance of type I collagen, fibronectin, and N-cadherin, and the appearance of tenascins, matrilins, and thrombospondins, including cartilage oligomeric protein, initiate the transition from chondroprogenitor cells to a fully committed chondrocyte.2,36–38 N-cadherin and N-CAM disappear in differentiating chondrocytes and are detectable later only in perichondrial cells. The differentiated chondrocytes can proliferate and undergo the complex process of hypertrophic maturation or remain within cartilage elements in articular joints.
Zwilling39 proposed that positional information for organization of the limb bud was impacted by diffusible agents generated at the tip of the limb bud and along its posterior margin, promoting the development of a cartilaginous anlage along proximal-distal and anterior-posterior axes. Limb buds develop from the lateral plate mesoderm.40 Patterning of limb mesenchyme is the result of interactions between the mesenchyme and the overlying epithelium.41 The embryonic limb possesses two signaling centers: the apical ectodermal ridge (AER) and the zone of polarizing activity (ZPA), which produce signals responsible for directing proximal-distal outgrowth (AER) and anterior-posterior patterning (ZPA).2,36
Much of our current understanding of limb development is based on early studies in chickens and more recently in mice. Regulatory events are controlled by interacting patterning systems involving FGF, hedgehog, BMP, and Wnt pathways, each of which functions sequentially over time (see Figure 1-3).40 Wnt signaling via β-catenin is required to induce FGFs, such as FGF-10 and FGF-8, which act in positive feedback loops.40,42 FGF-2, FGF-4, and FGF-8 (induced by Wnt-3A43), from specialized epithelial cells in the AER that are covering the limb bud tip, control proximal-distal (shoulder/finger) outgrowth.44 The homeobox (Hox) transcription factors encoded by HoxA and HoxD gene clusters, which are crucial for early events of limb patterning in the undifferentiated mesenchyme, are required for the expression of FGF-8 and Sonic hedgehog (Shh),45 and they modulate the proliferation of cells within the condensations.33 Among the Hox genes, Hoxa13 and Hoxd13 enhance and Hoxa11 and Hoxd11 suppress early events in the formation of cartilage anlagen.
Wnt-7A is expressed early during limb bud development, where it acts to maintain Shh expression.40 Shh, produced by a small group of cells in the posterior zone of the ZPA (in response to retinoic acid in the mesoderm46 and FGF-4 in the AER47), plays a key role in directing anterior-posterior (e.g., little finger/thumb) patterning46,48 and in stimulating expression of BMP-2, BMP-4, BMP-7, and Hox genes.49–51 Shh signaling, which is required for early limb patterning, but not for limb formation, is mediated by the Shh receptor Patched (Ptc1), which activates another transmembrane protein, Smoothened (Smo), and inhibits processing of the Gli3 transcription factor to a transcriptional repressor.42,52 Dorsal-ventral (e.g., knuckles/palm) patterning depends on secretion of Wnt-7A53 and expression of the following transcription factors: radical fringe (r-Fng) by the dorsal ectoderm, and engrailed (En-1) and Lmx1b (which is induced by Wnt-7A) by the ventral endoderm.42,54
BMP-2, BMP-4, and BMP-7 coordinately regulate the patterning of limb elements within condensations depending on the temporal and spatial expression of BMP receptors and BMP antagonists, such as noggin and chordin, as well as the availability of SMADs (signaling mammalian homologs of Drosophila mothers against decapentaplegic).40,55–57 In vitro and in vivo studies have shown that BMP signaling is required for the formation of precartilaginous condensations and for the differentiation of precursors into chondrocytes.58 Growth of the condensation ceases when noggin inhibits BMP signaling and permits overt differentiation to chondrocytes, which often are designated as chondroblasts. The cartilage formed serves as a template for formation of cartilage elements in the vertebra, sternum, and rib, and for limb elongation or endochondral bone formation.
Molecular Signals in Cartilage Morphogenesis and Growth Plate Development
The cartilage anlagen grow by cell division and deposition of the extracellular matrix and by apposition of proliferating cells from the inner chondrogenic layer of the perichondrium. The nuclear transcription factor, Sox9, is one of the earliest markers expressed in cells undergoing condensation and is required for the subsequent stage of chondrogenesis characterized by the deposition of matrix containing collagens II, IX, and XI and aggrecan in the cartilage anlagen.59,60 Two additional Sox family members, L-Sox5 and Sox6, which are not present in early mesenchymal condensations but are coexpressed with Sox9 during chondrocyte differentiation,61 have a high degree of sequence identity with each other but have no sequence homology with Sox9, except in the high-mobility group (HMG) box. They can form homodimers or heterodimers, which bind more efficiently to pairs of HMG box sites than to single sites, and in contrast to Sox9, they contain no transcriptional activation domain. The expression of SOX proteins depends on BMP signaling via BMPR1A and BMPR1B, which are functionally redundant and active in chondrocyte condensations but not in the perichondrium.58 L-Sox5 and Sox6 are required for the expression of Col9a1, aggrecan, link protein, and Col2a1 during overt chondrocyte differentiation.62 The runt domain transcription factor, Runx2 (also known as core binding factor, Cbfa1), is expressed in all condensations, including those that are destined to form bone.63–65
Throughout chondrogenesis, the balance of signaling by BMPs and FGFs determines the rate of proliferation while adjusting the pace of differentiation.10 In the long bones, long after condensation, BMP-2, BMP-3, BMP-4, BMP-5, and BMP-7 are expressed primarily in the perichondrium, and only BMP-7 is expressed in the proliferating chondrocytes.10 BMP-6 is found later exclusively in hypertrophic chondrocytes along with BMP-2. More than 23 FGFs have been identified so far.66 The specific ligands that activate each FGF receptor (R) during chondrogenesis in vivo have been difficult to identify because signaling depends on the temporal and spatial location of not only the ligands, but also the receptors.67 FGFR2 is upregulated early in condensing mesenchyme and is present later in the periphery of the condensation along with FGFR1, which is expressed in surrounding loose mesenchyme. FGFR3 is associated with proliferation of chondrocytes in the central core of the mesenchymal condensation and may overlap with FGFR2. Proliferation of chondrocytes in the embryonic and postnatal growth plate is regulated by multiple mitogenic stimuli, including FGFs, which converge on cyclin D1.68
In the growth plate, FGFR3 serves as a master inhibitor of chondrocyte proliferation via phosphorylation of the Stat1 transcription factor, which increases expression of the cell cycle inhibitor p21.69 More recent studies suggest that FGF-18 is the preferred ligand of FGFR3 because Fgf18-deficient mice have an expanded zone of proliferating chondrocytes similar to that in Fgfr3-deficient mice, and that FGF-18 can inhibit Indian hedgehog (Ihh) expression.70 FGF-18 and FGF-9 are expressed in the perichondrium and periosteum and form a functional gradient from the proximal proliferating zone, where FGF-18 acts via FGFR3 to downregulate proliferation and subsequent maturation.70,71 FGF-18 and FGF-9 interact with FGFR1 in the prehypertrophic and hypertrophic zones, where more recent evidence indicates that they regulate vascular invasion by inducing the expression of vascular endothelial growth factor (VEGF) and VEGFR1. As the epiphyseal growth plate develops, FGFR3 disappears, and FGFR1 expression is upregulated in prehypertrophic and hypertrophic chondrocytes, suggesting a role for FGFR1 in the regulation of cell survival and differentiation and possibly cell death.67
The proliferation of chondrocytes in the lower proliferative and prehypertrophic zones is under the control of a local negative feedback loop involving signaling by parathyroid hormone–related protein (PTHrP) and Ihh.72 Ihh expression is restricted to the prehypertrophic zone, and the PTHrP receptor is expressed in the distal zone of periarticular chondrocytes. Adjacent, surrounding perichondrial cells express the Hedgehog receptor patched (Ptc), which on Ihh binding, similar to Shh in the mesenchymal condensations, activates Smo and induces Gli transcription factors; this can feedback regulate Ihh target genes in a positive (Gli1 and Gli2) or negative (Gli3) manner.73 Ihh induces expression of PTHrP in the perichondrium,74 and PTHrP signaling stimulates cell proliferation via its receptor expressed in the periarticular chondrocytes.75 These interactions are modulated by a balance of BMP and FGF signaling that adjusts the pace of chondrocyte terminal differentiation to the proliferation rate.10 FGF-18 or FGFR3 signaling can inhibit Ihh expression,70 and BMP signaling upregulates the expression of Ihh in cells that are beyond the range of the PTHrP-induced signal.10 Evidence indicates that Ihh acts independently of PTHrP on periarticular chondrocytes to stimulate differentiation of columnar chondrocytes in the proliferative zone, whereas PTHrP acts by preventing premature differentiation into prehypertrophic and hypertrophic chondrocytes, suppressing premature expression of Ihh.76 Ihh and PTHrP, by transiently inducing proliferation markers and repressing differentiation markers, function in a temporospatial manner to determine the number of cells that remain in the chondrogenic lineage versus the number that enter the endochondral ossification pathway.72,77
Endochondral Ossification
The development of long bones from the cartilage anlagen occurs by a process termed endochondral ossification, which involves terminal differentiation of chondrocytes to the hypertrophic phenotype, cartilage matrix calcification, vascular invasion, and ossification (see Figure 1-4).28,77–79 This process is initiated when cells in the central region of the anlage begin to hypertrophy, increasing cellular fluid volume by almost 20 times. Ihh plays a pivotal role in regulating endochondral bone formation by synchronizing perichondrial maturation with chondrocyte hypertrophy, which is essential for initiating the process of vascular invasion.80 Ihh is expressed in prehypertrophic chondrocytes as they exit the proliferative phase and enter the hypertrophic phase, at which time they begin to express the hypertrophic chondrocyte marker, type X collagen (Col10a1), and alkaline phosphatase. These cells are responsible for laying down the cartilage matrix, which subsequently undergoes mineralization.
Runx2, which serves as a positive regulatory factor in chondrocyte maturation to hypertrophy,81 is expressed in the adjacent perichondrium and in prehypertrophic chondrocytes, but less in late hypertrophic chondrocytes,82,83 overlapping with Ihh, Col10a1, and BMP-6.77,84 BMP-induced Smad1 interacts with Runx2, and Runx2 and Smad1 are important for chondrocyte hypertrophy.81,85,86 An essential role for Runx2 in the process of chondrocyte hypertrophy is supported by the observation that terminal differentiation is blocked in Runx2-deficient mice.64,87 Interactions with components of the extracellular matrix also contribute to regulation of the process of chondrocyte terminal differentiation. Matrix metalloproteinase (MMP)-13, a downstream target of Runx2, is expressed by terminal hypertrophic chondrocytes,88–91 and MMP-13 deficiency results in significant interstitial collagen accumulation, leading to a delay in endochondral ossification in the growth plate with increased length of the hypertrophic zone.92,93
In contrast, Col10a1 knockout mice and transgenic mice with a dominant interference Col10a1 mutation have subtle growth plate phenotypes with compressed proliferative and hypertrophic zones and altered mineral deposition.94 Mutations in the COL10a1 gene are associated with the dwarfism observed in human chondrodysplasias. These mutations affect regions of the growth plate that are under great mechanical stress, and it has been suggested that the defect in skeletal growth may be due in part to alteration of the mechanical integrity of the pericellular matrix in the hypertrophic zone, although a role for defective vascularization also has been proposed.95 The extracellular matrix remodeling that accompanies chondrocyte terminal differentiation is thought to induce an alteration in the environmental stress experienced by hypertrophic chondrocytes, which eventually undergo apoptosis.77,96,97 Together these studies indicate that the composition and remodeling of the extracellular matrix play an important role in processes associated with chondrocyte hypertrophy, vascular invasion, and, as discussed subsequently, osteoblast recruitment and subsequent bone formation.90
Vascular invasion of the hypertrophic zone is required for the replacement of calcified cartilage by bone.84,91 The angiogenic factor, VEGF, promotes vascular invasion by specifically activating localized receptors, including Flk expressed in endothelial cells in the perichondrium or surrounding soft tissues, neuropilin 1 (Npn1) expressed in late hypertrophic chondrocytes, or Npn2 expressed exclusively in the perichondrium.28 VEGF is expressed as three different isoforms: VEGF188, a matrix-bound form, is essential for metaphyseal vascularization, whereas the soluble form, VEGF120 (VEGFA), regulates chondrocyte survival and epiphyseal cartilage angiogenesis.98–100 VEGF164 can be soluble or matrix bound and may act directly on chondrocytes via Npn2. VEGF is released from the extracellular matrix by MMPs, including MMP-9, membrane-type (MT)1-MMP (MMP-14), and MMP-13. MMP-9 is expressed by endothelial cells that migrate into the central region of the hypertrophic cartilage.90 MMP-14, which has a broader range of expression than MMP-9, is essential for chondrocyte proliferation and secondary ossification,101 whereas MMP-13 is found exclusively in late hypertrophic chondrocytes.82 These events of cartilage matrix remodeling and vascular invasion are required for the migration and differentiation of osteoclasts and osteoblasts, which remove the mineralized cartilage matrix and replace it with bone.
Development of the Joint Capsule and Synovium
The interzone and the contiguous perichondrial envelope, of which the interzone is a part, contain the mesenchymal cell precursors that give rise to other joint components, including the joint capsule, synovial lining, menisci, intracapsular ligaments, and tendons.3,4,102,103 The external mesenchymal tissue condenses as a fibrous capsule. The peripheral mesenchyme becomes vascularized and is incorporated as the synovial mesenchyme, which differentiates into a pseudomembrane at about the same time as cavitation begins in the central interzone (stage 23, approximately 8 weeks). The menisci arise from eccentric portions of the articular interzone. In common usage, the term synovium refers to the true synovial lining and the subjacent vascular and areolar tissue, up to—but excluding—the capsule. Synovial lining cells can be distinguished as soon as multiple cavities within the interzone begin to coalesce. At first, these are exclusively fibroblast-like (type B) cells.
As the joint cavity increases in size, synovial lining cell layers expand through proliferation of fibroblast-like cells and recruitment of macrophage-like (type A) cells from the circulation.104 The synovial lining cells express the hyaluronan receptor, CD44, and UDPGD, the levels of which remain elevated after cavitation. This increased activity likely contributes to the high concentration of hyaluronan in joint fluids.30,105 Further synovial expansion results in the appearance of synovial villi at the end of the second month, early in the fetal period, which greatly increases the surface area available for exchange between the joint cavity and the vascular space. Cadherin-11 is an additional molecule expressed by synovial lining cells.106,107 It is essential for establishment of synovial lining architecture during development, where its expression correlates with cell migration and tissue outgrowth of the synovial lining.
The role of innervation in the developing joint is not well understood. A dense capillary network develops in the subsynovial tissue, with numerous capillary loops that penetrate into the true synovial lining layer. The human synovial microvasculature is already innervated by 8 weeks (stage 23) of gestation, around the time of joint cavitation,102 as is shown by immunoreactivity for the neuronal “housekeeping” enzymes.108 Evidence of neurotransmitter function is not found until much later, however, with the appearance of the sensory neuropeptide, substance P, at 11 weeks. The putative sympathetic neurotransmitter, neuropeptide Y, appears at 13 weeks of gestation, along with the catecholamine-synthesizing enzyme tyrosine hydroxylase. The finding that the Slit2 gene, which functions for the guidance of neuronal axons and neurons, is expressed in the mesenchyme adjacent to the AER (stages 20 to 22) and in the peripheral mesenchyme of the limb bud (stages 23 to 28) suggests that innervation is an integral part of synovial joint development.109
Development of Nonarticular Joints
In contrast to articular joints, the temporomandibular joint develops slowly, with cavitation at a crown-rump length of 57 to 75 mm (i.e., well into the fetal stage).110 This slow development may occur because this joint develops in the absence of a continuous blastema and involves the insertion between bone ends of a fibrocartilaginous disk that arises from muscular and mesenchymal derivatives of the first pharyngeal arch.
The development of other types of joints, such as synarthroses, is similar to that of diarthrodial joints, except that cavitation does not occur and synovial mesenchyme is not formed. In these respects, synarthroses and amphiarthroses resemble the “fused” peripheral joints induced by paralyzing chicken embryos,111 and they may develop as they do because there is relatively little motion during their formation.
Human vertebrae and intervertebral disks develop as units, each derived from a homogeneous blastema arising from a somite. Each embryonic intervertebral disk serves as a rostral and caudal chondrogenic zone for the two adjacent evolving vertebral bodies. The periphery of the embryonic “disk” is replaced by the annulus fibrosus.112 The intervertebral disk bears many similarities to the joint; the annulus is the joint capsule, the nucleus pulposus is the joint cavity, and the vertebral end plates are the cartilage-covered bone ends composing the articulation. The proteoglycans and collagens expressed during development of the intervertebral disk have been mapped and reflect the complex structure-function relationships that allow flexibility and resistance to compression in the spine.113–116
Development of Articular Cartilage
In the vertebrate skeleton, cartilage is the product of cells from three distinct embryonic lineages. Craniofacial cartilage is formed from cranial neural crest cells, the cartilage of the axial skeleton (intervertebral disks, ribs, and sternum) forms from paraxial mesoderm (somites), and the articular cartilage of the limbs is derived from the lateral plate mesoderm.2 In the developing limb bud, mesenchymal condensations, followed by chondrocyte differentiation and maturation, occur in digital zones, whereas undifferentiated mesenchymal cells in the interdigital web zones undergo cell death.117 Embryonic cartilage is destined for one of several fates: It can remain as permanent cartilage, as on the articular surfaces of bones, or it can provide a template for the formation of bones by endochondral ossification. During development, chondrocyte maturation expands from the central site of the original condensation, which forms the cartilage anlage resembling the shape of the future bone, toward the ends of the forming bones. During joint cavitation, the peripheral interzone is absorbed into each adjacent cartilaginous zone, evolving into the articular surface. The articular surface is destined to become a specialized cartilaginous structure that does not normally undergo vascularization and ossification.
More recent evidence indicates that postnatal maturation of the articular cartilage involves an appositional growth mechanism originating from progenitor cells at the articular surface, rather than by an interstitial mechanism.114 The chondrocytes of mature articular cartilage are terminally differentiated cells that are capable of expressing cartilage-specific matrix molecules, such as type II collagen and aggrecan (see following section).19,21,24 Through the processes described previously, the articular joint spaces are developed and are lined on all surfaces by cartilage or by synovial lining cells. These two different tissues merge at the enthesis, the region at the periphery of the joint where the cartilage melds into bone, and where ligaments and the capsule are attached.118 In the postnatal growth plate, differentiation of the perichondrium also is linked to differentiation of the chondrocytes in the epiphysis to form the different zones of the growth plate, contributing to longitudinal bone growth.28,77
Organization and Physiology of the Mature Joint
The unique structural properties and biochemical components of diarthrodial joints make them extraordinarily durable load-bearing devices.119 The mature diarthrodial joint is a complex structure, influenced by its environment and by mechanical demands (see Chapter 6). Structural differences between joints are determined by their different functions. The shoulder joint, which demands an enormous range of motion, is stabilized primarily by muscles, whereas the hip, requiring motion and antigravity stability, has an intrinsically stable ball-and-socket configuration. Components of the “typical” synovial joint include synovium, muscles, tendons, ligaments, bursae, menisci, articular cartilage, and subchondral bone. The anatomy and physiology of muscles are described in detail in Chapter 5.
Synovium
The synovium lines the joint cavity and is the sight of production of synovial fluid that provides nutrition for the articular cartilage and lubricates the cartilage surfaces. It is a thin membrane between the fibrous joint capsule and the fluid-filled synovial cavity that attaches to skeletal tissues at the bone-cartilage interface and does not encroach on the surface of the articular cartilage. It is divided into functional compartments: the lining region (synovial intima), the subintimal stroma, and the neurovasculature (Figure 1-6). The synovial intima, also termed synovial lining, is the superficial layer of the normal synovium that is in contact with the intra-articular cavity.105,120 The synovial lining is loosely attached to the subintima, which contains blood vessels, lymphatics, and nerves. Capillaries and arterioles generally are located directly underneath the synovial intima, whereas venules are located closer to the joint capsule.
A transition from loose to dense connective tissue occurs from the joint cavity to the capsule. Most cells in the normal subintimal stroma are fibroblasts and macrophages, although adipocytes and occasional mast cells are present.105 These compartments are not circumscribed by basement membranes but nonetheless have distinct functions; they are separated from each other by chemical barriers, such as membrane peptidases, which limit the diffusion of regulatory factors between compartments. Synovial compartments are unevenly distributed within a single joint. Vascularity is high at the enthesis, where synovium, ligament, and cartilage coalesce.121 Far from being a homogeneous tissue in continuity with the synovial cavity, synovium is highly heterogeneous, and synovial fluid may be poorly representative of the tissue-fluid composition of any synovial tissue compartment. In rheumatoid arthritis, the synovial lining of diarthrodial joints is the site of the initial inflammatory process.122,123 This lesion is characterized by proliferation of synovial lining cells, increased vascularization, and infiltration of the tissue by inflammatory cells, including lymphocytes, plasma cells, and activated macrophages (see Chapter 53).124–126
Synovial Lining
The synovial lining, a specialized condensation of mesenchymal cells and extracellular matrix, is located between the synovial cavity and the stroma. In normal synovium, the lining layer is two to three cells deep, although intra-articular fat pads usually are covered by only a single layer of synovial cells, and ligaments and tendons are covered by synovial cells that are widely separated. At some sites, lining cells are absent, and extracellular connective tissue constitutes the lining layer.127 Such “bare areas” become increasingly frequent with advancing age.128 Although the synovial lining is often referred to as the synovial membrane, the term membrane is more correctly reserved for endothelial and epithelial tissues that have basement membranes, tight intercellular junctions, and desmosomes. Instead, synovial lining cells lie loosely in a bed of hyaluronate interspersed with collagen fibrils. This is the macromolecular sieve that imparts the semipermeable nature of the synovium. The absence of any true basement membrane is a major determinant of joint physiology.
Early electron microscopic studies characterized lining cells as macrophage-derived type A synoviocytes and fibroblast-derived type B synoviocytes.129 High UDPGD activity and CD55 are used to distinguish type B synovial cells, whereas nonspecific esterase and CD68 typify type A cells.130,131 Normal synovium is lined predominantly by fibroblast-like cells, whereas macrophage-like cells account for only 10% to 20% of lining cells (see Figure 1-6).
Type A, macrophage-like synovial cells contain vacuoles, a prominent Golgi apparatus, and filopodia, but they have little rough endoplasmic reticulum. These cells express numerous cell surface markers of the monocyte-macrophage lineage, including CD11b, CD68, CD14, CD163, and the immunoglobulin (Ig)G Fc receptor, FcγRIIIa.105 Synovial intimal macrophages are phagocytic and may provide a mechanism by which particulate matter can be cleared from the normal joint cavity. Similar to other tissue macrophages, these cells have little capacity to proliferate and are likely localized to the joint during development. The op/op osteopetrotic mouse that is deficient in macrophages because of an absence of macrophage colony-stimulating factor also lacks synovial macrophages.132 This finding provides further evidence that type A synovial cells are of a common lineage with other tissue macrophages. Although they represent only a small percentage of cells in the normal synovium, macrophages are recruited from the circulation during synovial inflammation, in part from subchondral bone marrow through vascular channels near the enthesis.
The type B, fibroblast-like synovial cell contains fewer vacuoles and filopodia than type A cells and has abundant protein-synthetic organelles. Similar to other fibroblasts, lining cells express the collagen synthesis enzyme and synthesize extracellular matrix components, including collagens, sulfated proteoglycans, fibronectin, fibrillin-1, and tenascin.105,133 They have the potential to proliferate, although proliferation markers are rarely seen in normal synovium.134 In contrast to stromal fibroblasts, synovial intimal fibroblasts express UDPGD and synthesize hyaluronan, an important constituent of synovial fluid.105 They also synthesize lubricin, which, together with hyaluronan, is necessary for the low-friction interaction of cartilage surfaces in the diarthrodial joint. Synovial lining cells bear abundant membrane peptidases on their surface, capable of degrading a wide range of regulatory peptides, such as substance P and angiotensin II.135 These enzymes may be important in limiting the diffusion of these potent peptide mediators away from the immediate vicinity of their site of release and action.
Normal synovial lining cells also express a rich array of adhesion molecules, including CD44, the principal receptor for hyaluronan; vascular cell adhesion molecule (VCAM)-1; and intercellular adhesion molecule (ICAM)-1.105,136–138 They are essential for cellular attachment to specific matrix components in the synovial lining region, preventing loss into the synovial cavity of cells subjected to deformation and shear stresses during joint movement. Adhesion molecules such as VCAM-1 and ICAM-1 potentially are involved in the recruitment of inflammatory cells during the evolution of arthritis. Cadherins mediate cell-cell adhesion between adjacent cells of the same type. The identification of cadherin-11 as a key adhesion molecule that regulates formation of the synovial lining during development and the synoviocyte function postnatally has provided the opportunity to examine its role in inflammatory joint disease.106 Recent studies have shown that cadherin-11 is highly expressed in fibroblast-like cells at the pannus-cartilage interface in rheumatoid synovium, where it plays a role in the invasive properties of the synovial fibroblasts139; treatment with a cadherin-11 antibody or a cadherin-11 fusion protein has been shown to reduce synovial inflammation and cartilage erosion in an animal model of arthritis.107
Synovial Vasculature
The subintimal synovium contains blood vessels, which provide the blood flow required for solute and gas exchange in the synovium itself and for generation of synovial fluid.121 The avascular articular cartilage also depends on nutrition in the synovial fluid, derived from the synovial vasculature. The vascularized synovium behaves similar to an endocrine organ, generating factors that regulate synoviocyte function and serving as a selective gateway that recruits cells from the circulation during stress and inflammation.140 Finally, synovial blood flow plays an important role in regulating intra-articular temperature.
The synovial vasculature can be divided, on morphologic and functional grounds, into arterioles, capillaries, and venules. In addition, lymphatics accompany arterioles and larger venules.105,121 Arterial and venous networks of the joint are complex and are characterized by arteriovenous anastomoses that communicate freely with blood vessels in periosteum and periarticular bone. As large synovial arteries enter the deep layers of the synovium near the capsule, they give off branches, which bifurcate again to form microvascular units in the subsynovial layers. The synovial lining region, the surfaces of intra-articular ligaments, and the entheses (in the angle of ligamentous insertions into bone) are particularly well vascularized.121
The distribution of synovial vessels, which were formed largely as a result of vasculogenesis during development of the joint, displays considerable plasticity. Vasculogenesis is a dynamic process that depends on cellular interactions with regulatory factors and the extracellular matrix that are also important in angiogenesis. In inflammatory arthritis, the density of blood vessels decreases relative to the growing synovial mass, creating a hypoxic and acidotic environment.141,142 Angiogenic factors such as VEGF, acting via VEGF receptors 1 and 2 (Flt-1 and Flk-1), and basic FGF promote proliferation and migration of endothelial cells—a process that is facilitated by matrix-degrading enzymes and adhesion molecules such as integrin αvβ3 and E-selectin, expressed by activated endothelial cells.143–145 Vessel maturation is facilitated by angiopoietin-1 acting via the Tie-2 receptor. Angiogenic molecules are restricted to the capillary epithelium in normal synovium, but their levels are elevated in inflamed synovium in perivascular sites and areas remote from vessels.146,147
Regulation of Synovial Blood Flow
Synovial blood flow is regulated by intrinsic (autocrine and paracrine) and extrinsic (neural and humoral) systems. Locally generated factors, such as the peptide vasoconstrictors angiotensin II and endothelin-1, act on adjacent arteriolar smooth muscle to regulate regional vascular tone.121 Normal synovial arterioles are richly innervated by sympathetic nerves containing vasoconstrictors, such as norepinephrine and neuropeptide Y, and by “sensory” nerves that also play an efferent vasodilatory role by releasing neuropeptides, such as substance P and calcitonin gene–related peptide.148,149 Arterioles regulate regional blood flow. Capillaries and postcapillary venules are sites of fluid and cellular exchange. Correspondingly, regulatory systems are differentially distributed along the vascular axis. Angiotensin-converting enzyme, which generates angiotensin II, is localized predominantly in arteriolar and capillary endothelia and is decreased during inflammation.150 Specific receptors for angiotensin II and for substance P are abundant on synovial capillaries, with lower densities on adjacent arterioles. Dipeptidyl peptidase IV, a peptide-degrading enzyme, is specifically localized to the cell membranes of venular endothelium. The synovial vasculature not only is functionally compartmentalized from the surrounding stroma, but is also highly specialized along its arteriovenous axis. Other unique characteristics of the normal synovial vasculature include the presence of inducible nitric oxidase synthase–independent 3-nitrotyrosine, a reaction product of peroxynitrite,151 and localization of the synoviocyte-derived CXCL12 chemokine on heparan sulfate receptors on endothelial cells,152 suggesting physiologic roles for these molecules in normal vascular function.
Joint Innervation
Dissection studies have shown that each joint has a dual nerve supply, consisting of specific articular nerves that penetrate the capsule as independent branches of adjacent peripheral nerves and articular branches that arise from related muscle nerves. The definition of joint position and the detection of joint motion are monitored separately and through a combination of multiple inputs from different receptors in varied systems. Nerve endings in muscle and skin and in the joint capsule mediate the sensation of joint position and movement.153,154 Normal joints have afferent (sensory) and efferent (motor) innervations. Fast-conducting, myelinated A fibers innervating the joint capsule are important for proprioception and detection of joint movement; slow-conducting, unmyelinated C fibers transmit diffuse pain sensation and regulate synovial microvascular function.
Normal synovium is richly innervated by fine, unmyelinated nerve fibers that follow the courses of blood vessels and extend into the synovial lining layers.148 These nerve fibers do not have specialized endings and are slow-conducting fibers; they may transmit diffuse, burning, or aching pain sensation. Sympathetic nerve fibers surround blood vessels, particularly in the deeper regions of normal synovium. They contain and release classic neurotransmitters, such as norepinephrine, and neuropeptides that constrict synovial blood vessels. Neuropeptides that are markers of sensory nerves include substance P, calcitonin gene–related peptide, neuropeptide Y, and vasoactive intestinal peptide.148,155–157
Afferent nerves containing substance P also have an efferent role in the synovium. Substance P is released from peripheral nerve terminals into the joint, and specific, G protein–coupled receptors for substance P are localized to microvascular endothelium in normal synovium. Abnormalities of articular innervation that are associated with inflammatory arthritis may contribute to the failure of synovial inflammation to resolve.148,158 Excessive local neuropeptide release may result in loss of nerve fibers owing to neuropeptide depletion. Synovial tissue proliferation without concomitant growth of new nerve fibers may lead to an apparent partial denervation of synovium.148,158 Studies in patients suggest that free nerve endings containing substance P may modulate inflammation and the pain pathway in osteoarthritis.159 Afferent nerve fibers from the joint play an important role in the reflex inhibition of muscle contraction. Trophic factors generated by motor neurons, such as the neuropeptide calcitonin gene–related peptide, are important in maintaining muscle bulk and a functional neuromuscular junction.160 Decreases in motor neuron trophic support during articular inflammation probably contribute to muscle wasting.
Mechanisms of joint pain have been reviewed in detail.161,162 In a noninflamed joint, most sensory nerve fibers do not respond to movement within the normal range; these are referred to as silent nociceptors. In an acutely inflamed joint, however, these nerve fibers become sensitized by mediators, such as bradykinin, neurokinin 1, and prostaglandins (peripheral sensitization), such that normal movements induce pain. Pain sensation is upregulated or downregulated further in the central nervous system, at the level of the spinal cord, and in the brain by central sensitization and gating of nociceptive input. Although the normal joint may respond predictably to painful stimuli, poor correlation has been noted between apparent joint disease and perceived pain in chronic arthritis. Pain associated with joint movements within the normal range is a characteristic symptom described by patients with chronically inflamed joints caused by rheumatoid arthritis. Chronically inflamed joints may not be painful at rest, however, unless acutely inflamed.163
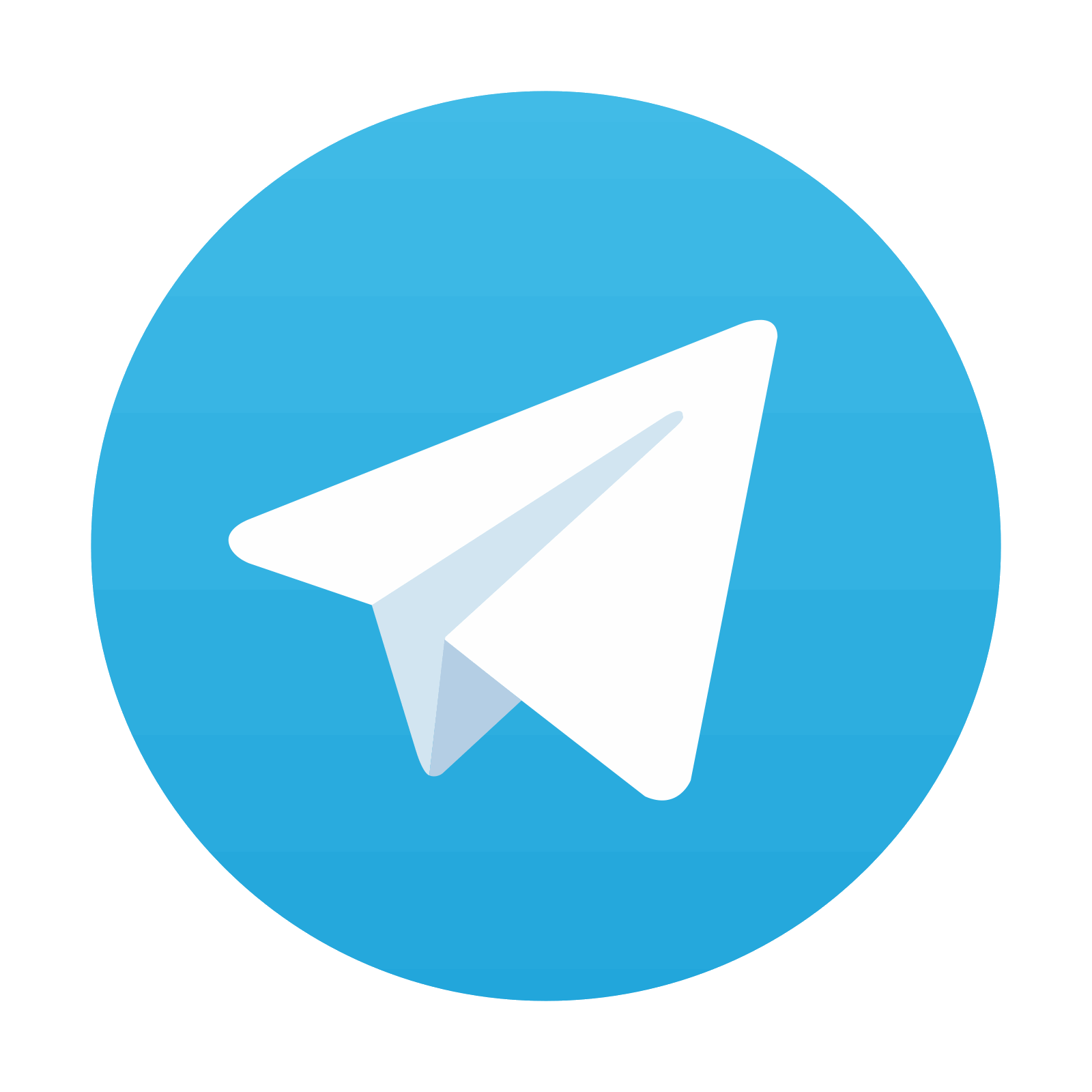
Stay updated, free articles. Join our Telegram channel
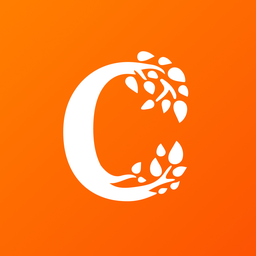
Full access? Get Clinical Tree
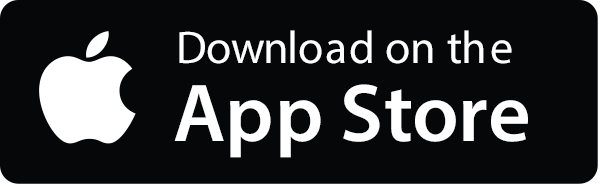
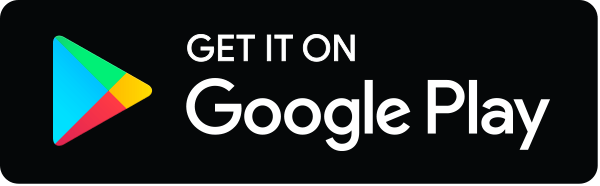