Assessment of Human Muscle Function
Walter R. Frontera
Jan Lexell
To move is all mankind can do, and for such the sole executant is muscle.
–Sir Charles Sherrington, Edinburgh, 1937-1938
The relevance of skeletal muscle to the performance of all types of physical activities (i.e., therapeutic, recreational, occupational, and others) and the successful participation in daily life and societal obligations should be well appreciated by all those who work in rehabilitation. Skeletal muscle plays an important role, primary and/or secondary, in the pathophysiology of many diseases, and skeletal muscle function is key to defining the nature and extent of impairments and activity limitations. Thus, understanding how to measure skeletal muscle function and how to interpret the results of various physiologic and functional tests is a necessary component of the education of all physiatrists and rehabilitation professionals. It is worth noting that this understanding is of special value to the advancement of research in the rehabilitation sciences because many biological and functional outcome variables used in scientific studies are directly associated with the function and structure of skeletal muscle. Finally, this chapter discusses this topic in the context of what is known about human skeletal muscle in health and disease. We will not review the extensive literature on muscle function based on studies in various animal models. With very few exceptions, the references will be those from human studies.
WHY MUSCLE?
The main function of the approximately 600 muscles in the human body is to convert chemical energy (i.e., fat and carbohydrates) into mechanical energy and thereby generate force. This force is transmitted from the active muscle fibers to the tendons with the help of the sarcolemma, special extracellular protein complexes, and connective tissue elements. The action of the tendons on bony structures results in the conversion of the force into joint and limb movement and displacement of individual body parts or the body as a unit. In principle, force generation can occur during brief moments, resulting in what is generally referred to as muscle strength, or force generation can be maintained over a period of time referred to as muscle endurance. In the clinical setting, the failure to generate force during a brief moment is what we generally call muscle weakness, as opposed to the inability to maintain force, which we refer to as muscle fatigue.
Skeletal muscle comprises 40% to 45% of the total body mass (1, 2, 3), and 55% of total muscle mass is distributed in the lower limbs. Muscle contains approximately 50% of the total body protein (4), and protein turnover in muscle represents 25% of the total body protein turnover (3, 5). More than half of the protein in muscle is found in the thick (myosin) and thin (actin) contractile filaments that generate and regulate force production (4, 6). Actin and myosin account for more than 80% of the protein in the myofibrillar complex. In addition to force generation, skeletal muscles contribute to basal metabolism; produce heat to maintain core temperature; regulate blood glucose; serve as storage for carbohydrates, lipids, and amino acids; contribute to energy production during exercise; and protect internal organs (7).
During illness, nitrogen must be mobilized from muscle to provide amino acids to the immune system, liver, and other organs. Thus, if sufficient nitrogen is not available due to muscle wasting associated with aging, immobilization, or severe illness, the body’s capacity to withstand an acute insult declines. The relationship between muscle function, illness, morbidity, and mortality is more obvious if one considers that morbidity becomes demonstrable at a 5% loss of lean mass and that the loss of 40% of lean body mass (LBM) is fatal (8). Finally, the extensive and considerable plasticity demonstrated by skeletal muscle under various conditions and in response to environmental influences such as bed rest, exercise training, and electrical stimulation makes it an ideal target for therapeutic and rehabilitative interventions.
From a functional point of view, skeletal muscle strength has been associated with comfortable and maximal walking speed (9, 10), the incidence and prevalence of disability (11, 12, 13), balance (12), time to rise from a chair (14, 15), ability to climb stairs (16), incidence of falls (17), and survival rate (18, 19). Muscle power, a related but distinct property of skeletal muscle, also shows a positive and significant association with functional status (20). This evidence provides strong support to the conclusion that enhancing and maintaining muscle strength and muscle endurance throughout the life span, whether it is through prevention or rehabilitation, may reduce the prevalence of limitations in recreational, household, daily, and personal care activities, both in health and disease (21).
MUSCLE ACTIONS AND UNITS OF MEASUREMENT
The need to use consistent terminology, definitions, and measurement units across research studies, in educational programs, and in clinical rehabilitation is of great importance. Furthermore, measuring devices must be reliable and valid indicators of muscle function. Muscle function and structure can be quantified using the International System of Units, a refinement of the metric system (22, 23, 24) (Table 3-1).
All types of muscle actions result in the production of force or torque (tendency of a force to produce rotation about an axis). When the force is applied against an immovable object and there is no joint angular movement, the action is called static (isometric). Work is defined as the product of force × distance, and power as the ratio of work over time. Therefore, by definition, because during a static muscle action the distance is zero, no work is performed and no power is produced.
When the muscle action results in the displacement of a given mass or body part at the same time that the origin and insertion of a muscle move closer together, the action is called dynamic (isotonic) concentric or shortening action. When the action results in the displacement of a given mass, and the origin and insertion of a muscle move further apart, the action is called dynamic eccentric or lengthening action. Both concentric and eccentric muscle actions result in work (force × distance), positive in the first case and negative in the latter. During many natural activities such as walking and running, concentric muscle actions occur in immediate combination with eccentric actions and are referred to as the stretch-shortening cycle (25, 26).
In these activities such as jumping and running upstairs, the rate at which force is developed is more important than generating maximal force. Thus, power ([work/time] or [force × velocity]) and not strength becomes the limiting factor. Some of the most important physical and biomechanical concepts in the study and measurement of muscle function are illustrated in Figure 3-1 (27).
Isokinetic muscle actions are dynamic and could be concentric or eccentric. This kind of muscle action is characterized by a combination of constant angular velocity and variable resistance. The resistance generated by the isokinetic device varies throughout the range of motion in order to match the torque generated by the muscle at each angle of the range of motion. It should be recognized that isokinetic actions represent an artificial situation that does not usually occur in nature outside of a laboratory. Many devices have been developed to measure muscle torque, work, power, and endurance based on the isokinetic concept. Isokinetic dynamometers, although expensive, are found in many research laboratories as well as in rehabilitation clinics (Fig. 3-2). Advantages of these devices
include the objective quantification of muscle function, the immediate availability of reports, the provision of feedback to the patient, their high reproducibility, and the opportunity to standardize sequential testing for follow-up purposes during the rehabilitation process. Disadvantages include the cost, lack of portability, and limited specificity in relation to muscle actions typical of daily activities.
include the objective quantification of muscle function, the immediate availability of reports, the provision of feedback to the patient, their high reproducibility, and the opportunity to standardize sequential testing for follow-up purposes during the rehabilitation process. Disadvantages include the cost, lack of portability, and limited specificity in relation to muscle actions typical of daily activities.
TABLE 3.1 Examples of Units for Assessing Muscle Structure and Function | ||||||||||||||||||||||
---|---|---|---|---|---|---|---|---|---|---|---|---|---|---|---|---|---|---|---|---|---|---|
|
![]() FIGURE 3-1. Physical and biomechanical concepts relevant to the assessment of human skeletal muscle function. Note the relevance of time on several variables, such as displacement, velocity, and work. Also, note the sequence of formulas leading from mass to power (27). |
![]() FIGURE 3-2. Picture of an isokinetic dynamometer. Here, the torque of the ankle dorsiflexor muscles is measured. |
The terms open kinetic chain and closed kinetic chain are used to describe two forms of muscle contractions and movements. The kinetic chain is a concept that describes a body segment as a series of mobile segments and linkages (28, 29, 30). In the case of the lower extremities, this chain allows forward propulsion during gait. When the foot is in contact with the ground, the kinetic chain is considered to be closed. When the foot is off the ground, the chain is said to be open. Examples of open kinetic chain exercises used in rehabilitation programs are leg extension, leg curls, arm curls, and bench press exercises. Exercises such as leg press, squats, and push-ups are examples of closed kinetic chain exercises. Closed kinetic chain exercises tend to activate agonist and antagonist muscle groups simultaneously (e.g., the knee extensors and flexors during squat exercises) and tend to be more functional (31). Both types of exercises could result in significant functional improvements after reconstruction of the anterior cruciate ligament (30).
Energetics of Muscle Actions
The energy needed for the muscle to perform its mechanical functions is supplied by three different energy-producing biochemical pathways (Fig. 3-3). The relative contribution of each pathway is determined by the duration and intensity of the muscle actions. Performance of a particular task is determined not only by the integrity and capacity of the sarcomeric proteins but also by the ability of these pathways to supply adenosine triphosphate (ATP). Thus, the results of the functional tests discussed later could be used as indicators of the status of the biochemical pathways. The low strength and/or endurance performance scores in patients with various neuromuscular diseases may relate to abnormalities in these pathways.
![]() FIGURE 3-3. Schematic drawing of the three different biochemical pathways for the energy production in skeletal muscles. |
In general, short-duration tasks lasting up to 10 seconds depend on existing stores of ATP and creatine phosphate (CP) (32, 33). These two stores are readily available and therefore could be used instantaneously. However, from a quantitative point of view, these ATP and CP stores are very small and have a limited ability to sustain muscle performance over time. Activities lasting between 10 seconds and 2 minutes are driven by the process of anaerobic glycolysis fueled by the transport of glucose into the muscle cell or the breakdown (glycogenolysis) of intramuscular carbohydrates (34, 35, 36, 37, 38). Finally, the energy for activities lasting more than 2 minutes is supplied mainly by the oxidative pathways in the mitochondria (Fig. 3-4). The fuel for these pathways can be derived from the end product of anaerobic glycolysis, circulating fatty acids, or intramuscular lipid stores (39, 40).
In real life, these biochemical processes combine in various proportions to provide ATP during physical activity and exercise. Activities can be classified as “predominantly” dependent on a particular pathway, since very few activities can be considered purely dependent on any given pathway.
In other words, a given activity may require a combination of all three processes, depending on fluctuations in the intensity of the exercise. For example, when a person is walking on a level at a comfortable speed, ATP supply may depend predominantly on oxidative pathways. Confronted with an incline or hill, the contribution of the glycolytic pathways increases. Another example in a different type of activity is the sprint at the end of a marathon race requiring the activation of the glycolytic pathway in a predominantly oxidative event.
In other words, a given activity may require a combination of all three processes, depending on fluctuations in the intensity of the exercise. For example, when a person is walking on a level at a comfortable speed, ATP supply may depend predominantly on oxidative pathways. Confronted with an incline or hill, the contribution of the glycolytic pathways increases. Another example in a different type of activity is the sprint at the end of a marathon race requiring the activation of the glycolytic pathway in a predominantly oxidative event.
![]() FIGURE 3-4. Sequence of activation of energy-supplying biochemical pathways in relation to the duration of physical activity. More than one pathway may be active at a given point in time. At the point of transition (e.g., 10 seconds, 2 minutes) there is significant overlap among systems. Total energy output per unit time (power) declines over time as the oxidative pathways become the predominant source of ATP. The transitions do not have to be unidirectional, since, for example, activation of the glycolytic pathways may be necessary at a particular point in time during the performance of a predominantly oxidative activity (46). |
Functional Characteristics of Skeletal Muscle
Muscle Strength
Muscle strength can be defined as the maximal force (or torque) generated by a muscle or muscle group at a specified velocity. Because strength depends on force production, it is generally measured in newtons (N) or newton-meters (Nm) in the case of torque. When reporting measurements of muscle strength, the type of muscle action must be stated (24). In other words, strength can be static (at different joint angles), dynamic (concentric or eccentric), or isokinetic (at different angular velocities).
It should be clear that there is no single strength measurement and that different kinds of strength can be expressed. Furthermore, under static conditions, force is influenced by fiber (and sarcomere) length (41) and mechanical leverage. Moreover, under dynamic conditions, the level of force is influenced by the velocity of the movement (41). These relationships are two of the most fundamental biologic properties of skeletal muscle and must be understood to appreciate the meaning of the results of functional tests. For example, the patient’s performance during a manual muscle test will not be reliable unless the test is always done at the same joint angle.
The force-length relationship illustrates how the sarcomere length, which defines the degree of overlap between actin and myosin and the formation of cross-bridges, determines force (Fig. 3-5). The optimal sarcomere length varies with the type of activity. For example, the optimal sarcomere length has been reported to be in the region around the plateau for ankle bending (42), walking (43), and jumping (44) activities and in the descending limb for slow pedaling (45). On the other hand, the force-velocity curve demonstrates a gradient of strength that ranges from the highest level during fast eccentric actions to the lowest level during fast concentric actions (Fig. 3-6). Static actions generate more than dynamic concentric actions but less force than dynamic eccentric actions, independent of the velocity.
Testing of Muscle Strength
Various methods and devices are used to measure the different types of muscle strength (46). These methods used in clinical practice and research require a maximal voluntary action on the part of the patient or volunteer. This is dependent on the ability of higher central nervous centers to recruit and modulate the frequency of discharge of the appropriate spinal motoneuron pool. The implication is that all those factors that influence the activation of the neuromuscular system such as age, various disorders in the central and peripheral nervous system, presence of pain, joint swelling, medications, fear and anxiety over the test, lack of motivation, time of the day, and environmental conditions such as noise may have a significant effect on strength measurements and should be controlled for
or factored into the analysis of the results (47, 48, 49). Needless to say, testing conditions must be standardized as much as possible, the same device and/or method and testing protocol must be used when repeated measurements are required, the subject must be encouraged to make a maximal effort during the test, and the presence of symptoms such as pain should be considered when interpreting the measurements. Furthermore, for comparisons among groups, it may be necessary to adjust for differences in muscle/body size using statistical techniques (50). Even under optimal conditions, a valid and reliable level may require that the strength test be repeated more than once (51). Over the last decade, there has been a growing interest in, and a gradual development of, the statistical methods for the analysis of reliability. Today, there is a general agreement that a comprehensive set of several statistical methods are required to fully address the reliability of a measurement method (52).
or factored into the analysis of the results (47, 48, 49). Needless to say, testing conditions must be standardized as much as possible, the same device and/or method and testing protocol must be used when repeated measurements are required, the subject must be encouraged to make a maximal effort during the test, and the presence of symptoms such as pain should be considered when interpreting the measurements. Furthermore, for comparisons among groups, it may be necessary to adjust for differences in muscle/body size using statistical techniques (50). Even under optimal conditions, a valid and reliable level may require that the strength test be repeated more than once (51). Over the last decade, there has been a growing interest in, and a gradual development of, the statistical methods for the analysis of reliability. Today, there is a general agreement that a comprehensive set of several statistical methods are required to fully address the reliability of a measurement method (52).
![]() FIGURE 3-5. Force-length relationship of skeletal muscle. Insets show schematic representations of cross-bridges. An optimal length results in the largest number of actin-myosin cross-bridges (plateau). When the muscle (or sarcomere) has been stretched too much (descending limb; sarcomere length >3.0 μm), no active force is produced by the actin-myosin cross-bridges. However, a level of force can be recorded as a result of the contribution of the passive elastic elements, including cytoskeletal proteins such as titin and nebulin and components of the sarcolemma. During the ascending limb (sarcomere length <1.75 μm), overlap of myofilaments interferes with actin-myosin cross-bridge formation (41). |
![]() FIGURE 3-6. Force-velocity relationship of skeletal muscle. Insets show schematic representations of actin-myosin cross-bridges. Static (isometric) strength (relative shortening velocity = 0) is higher than force at any given velocity of movement during concentric (shortening) muscle actions. On the other hand, eccentric (lengthening) muscle actions at any given velocity generate higher forces than static actions (41). |
Manual Muscle Testing
The method of muscle strength measurement used most frequently in the busy clinical setting is manual muscle testing. This technique uses a subjective scale (Table 3-2) that ranges from zero (complete paralysis) to normal strength (erroneously called normal muscle power by some authors) and is generally known as the “Classification of the Medical Research Council of Great Britain” (53, 54). The tester’s perception of the strength of a given muscle is influenced by the duration of the tester’s effort and the force applied during the test (55). The manual muscle testing scale is characterized by a fairly high level of intra- and interrater variability that limits its usefulness for research studies and clinical follow-up (56). To distinguish among the various degrees of muscle strength within a given level, this scale has been modified with the addition of intermediate levels (e.g., 4+ and 4-). Although clinically useful, there is no evidence that this modification increases the validity or the reliability of the method. It should be understood that this method represents an estimate of static strength at the tested joint angle. Extrapolation of the results to other joint angles and especially to dynamic actions must be done with great caution.
Static (Isometric) Maximal Voluntary Contraction
A static maximal voluntary contraction (MVC) refers to a condition in which a person attempts to recruit as many muscle fibers in a muscle as possible for the purpose of developing force (24). Although the need for a maximal voluntary effort applies to all forms of strength testing, the term maximal voluntary contraction is frequently associated with static strength testing (57). Devices such as hand-held dynamometers, cable tensiometers, force transducers, and isokinetic (angular velocity set at zero) dynamometers can be used to measure static muscle strength. Several studies (58, 59, 60) have shown good intra- and interrater reliability for the hand-held dynamometer in various patient populations. The simplicity and portability of these devices make it an attractive clinical instrument.
TABLE 3.2 Scale for Manual Muscle Testing | ||||||||||||||||||||||||||||
---|---|---|---|---|---|---|---|---|---|---|---|---|---|---|---|---|---|---|---|---|---|---|---|---|---|---|---|---|
|
The validity of the strength measurement depends on the activation level of the nervous system. Merton (61) introduced the use of electrical stimulation superimposed on a static maximal effort in an attempt to activate directly those motor units and muscle fibers not stimulated by the voluntary effort of the subject. The stimulus is applied to the motor nerve during the MVC, and if the force increases, it indicates a suboptimal activation of motor units by the central nervous system. This is often referred to as central activation failure (CAF) (62). In many studies, single impulse stimulation has been used to detect CAF. It has been shown that high-frequency maximal train stimulation may improve the detection of CAF during static (isometric) knee extensions (57, 62). This may be important in the clinical assessment of weakness, as it may distinguish weakness caused by CAF from that due to muscle wasting. This, in turn, could have very important implications for the design and evaluation of effective muscle-strengthening exercise therapy. The simultaneous assessment of CAF and muscle mass is advantageous and will facilitate the identification of the mechanism underlying muscle weakness in a particular patient. It is generally considered that healthy men and women even above the age of 70 have the ability to fully activate their muscles during an MVC (63, 64).
Repetition Maximum
During the course of physical rehabilitation and research studies involving exercise training, muscle strength is frequently measured using the one repetition maximum (1 RM) method. In the case of the extensors of the knee, DeLorme (65) defined the 1 RM as the maximum weight that can be lifted with one repetition with the knee going into complete
extension. The proper unit of strength measurement is the Newton (N), but in this test, strength is commonly expressed as the mass in kilograms (kg) of the lifted load. This is a simple, valid, and reliable method that uses relatively inexpensive equipment and has been shown to be safe even in the elderly population (66). One drawback is that, by definition, it requires full active range of motion, a condition that some patients with joint pain, swelling, or contractures may be unable to satisfy. Also, if the test is not properly performed and too many repetitions are used to determine the 1 RM, muscle fatigue may interfere with the subject’s ability to generate maximal force.
extension. The proper unit of strength measurement is the Newton (N), but in this test, strength is commonly expressed as the mass in kilograms (kg) of the lifted load. This is a simple, valid, and reliable method that uses relatively inexpensive equipment and has been shown to be safe even in the elderly population (66). One drawback is that, by definition, it requires full active range of motion, a condition that some patients with joint pain, swelling, or contractures may be unable to satisfy. Also, if the test is not properly performed and too many repetitions are used to determine the 1 RM, muscle fatigue may interfere with the subject’s ability to generate maximal force.
![]() FIGURE 3-7. Theoretical repetition maximum (RM) continuum. Note the relationship between the number of repetitions and the specific muscle physiologic characteristic affected by training at the specific level (67). |
The concept of a continuum of repetition is frequently used when designing strength-conditioning programs (67). The RM refers to the exact resistance that allows a specific number of repetitions to be performed. Figure 3-7 shows the relationship between RM, number of repetitions, and muscle physiologic characteristic affected by training at a specific level.
Isokinetic Strength
A number of isokinetic devices have been used to measure muscle strength in research laboratories and rehabilitation clinics (see Fig. 3-2). Although several muscle groups have been studied with these devices, most of the information concerns the knee, ankle (Fig. 3-8), and shoulder muscle groups. The lever arm is aligned with the axis of rotation of the joint to be tested, and proximal and distal segments are stabilized using Velcro straps limiting the contribution of agonists. The tester sets the angular velocity (available range 0 to 450 degrees per second or 0 to 7.9 rad per second), and the research subjects or patients are usually asked to complete three to five maximal repetitions, with the maximal torque used as a measurement of strength.
Individual brands of isokinetic dynamometers have been shown to be reliable (48, 49, 51, 68, 69, 70), including measurements of isokinetic eccentric strength (49, 69) and strength in patients with Duchenne or Becker muscular dystrophies (71) or stroke (72). Reliability is usually best at low angular velocities and gradually decreases with increased angular velocity. At very high velocities (e.g., above 180 degrees per second), reliability is often considered poor. The comparison of strength values among the different brands of isokinetic dynamometers appears to be less valid. The spectrum of indicators of muscle function provided includes measurements of muscle work (integrated area under the curve), endurance (see later), agonists/antagonists ratios, and differences between sides that can be used to assess for muscle imbalances and asymmetries. On the other hand, the devices are expensive, the isokinetic nature of the muscle action does not allow for direct comparisons with daily activities, and the range of angular velocities does not extend to the speed of many sports and daily actions.
Finally, measurements of isokinetic strength at various angular velocities have been used to study, in vivo, the whole muscle torque-velocity curve and the effects of strength training on muscle contractile behavior (73, 74, 75). These studies require the use of torque at multiple given joint angles rather than peak torque over the continuum of available angles in order to control for the effect of muscle length on force development.
Muscle Power
Leg extensor power could be a very important measurement in sports as well as in rehabilitation because power is more relevant for time-dependent/time-critical activities than strength. Power has been shown to correlate with gait speed, time to rise from a chair, stair-climb time, and self-reported disability (76, 77). In addition, power declines with aging at a faster rate than strength (78). The maximum leg extension power output produced by both legs and each leg separately can be estimated using a power rig bench or resistance training machines. The power rig bench measures force and velocity
of leg movement, whereas the test using resistance training machines is based on the performance of one repetition at a percentage of the 1 RM. Peak power has been reported to occur usually at 70% of the 1 RM using the resistance training machines (20). Several test trials are usually performed with a 45- to 60-second rest between trials and the maximum value recorded. The coefficient of variation of this method is 6% (79).
of leg movement, whereas the test using resistance training machines is based on the performance of one repetition at a percentage of the 1 RM. Peak power has been reported to occur usually at 70% of the 1 RM using the resistance training machines (20). Several test trials are usually performed with a 45- to 60-second rest between trials and the maximum value recorded. The coefficient of variation of this method is 6% (79).
Other investigators have designed power tests that combine an eccentric muscle action followed by a concentric action of the same muscle group with a very brief static action in between (80). This is a test of the stretch-shortening cycle (81). A mat or platform that is connected to a timer is used to measure flight time (time spent off the ground) during a vertical jump from which jump height can be calculated. The jump is preceded by a countermovement or semisquat position. The output is fed into a computer for analysis and calculation of muscle power.
Muscle Endurance (and Fatigue)
The definitions of endurance and fatigue vary with the source. For the purpose of this chapter, endurance will be defined as the “time limit of a person’s ability to maintain either an isometric force or a power level involving combinations of concentric and/or eccentric muscular actions” (24). Endurance can be measured in seconds. Fatigue, on the other hand, is the inability to maintain a given level of force output (82). Alternatively, neuromuscular fatigue can be defined as “any reduction in the force-generating capacity of the total neuromuscular system” and can be due to factors that affect muscle fibers, the neuromuscular junction, and/or the nervous system (83). In humans, fatigue results in the loss of voluntary force and power production (84), a reduction in electrically stimulated maximal force and the maximum rate of force development (85), altered recruitment pattern of muscles (86), impaired neuromuscular performance in activities such as jumping (87), and even a reduction in sensory function such as the acuity of the joint movement sense (88
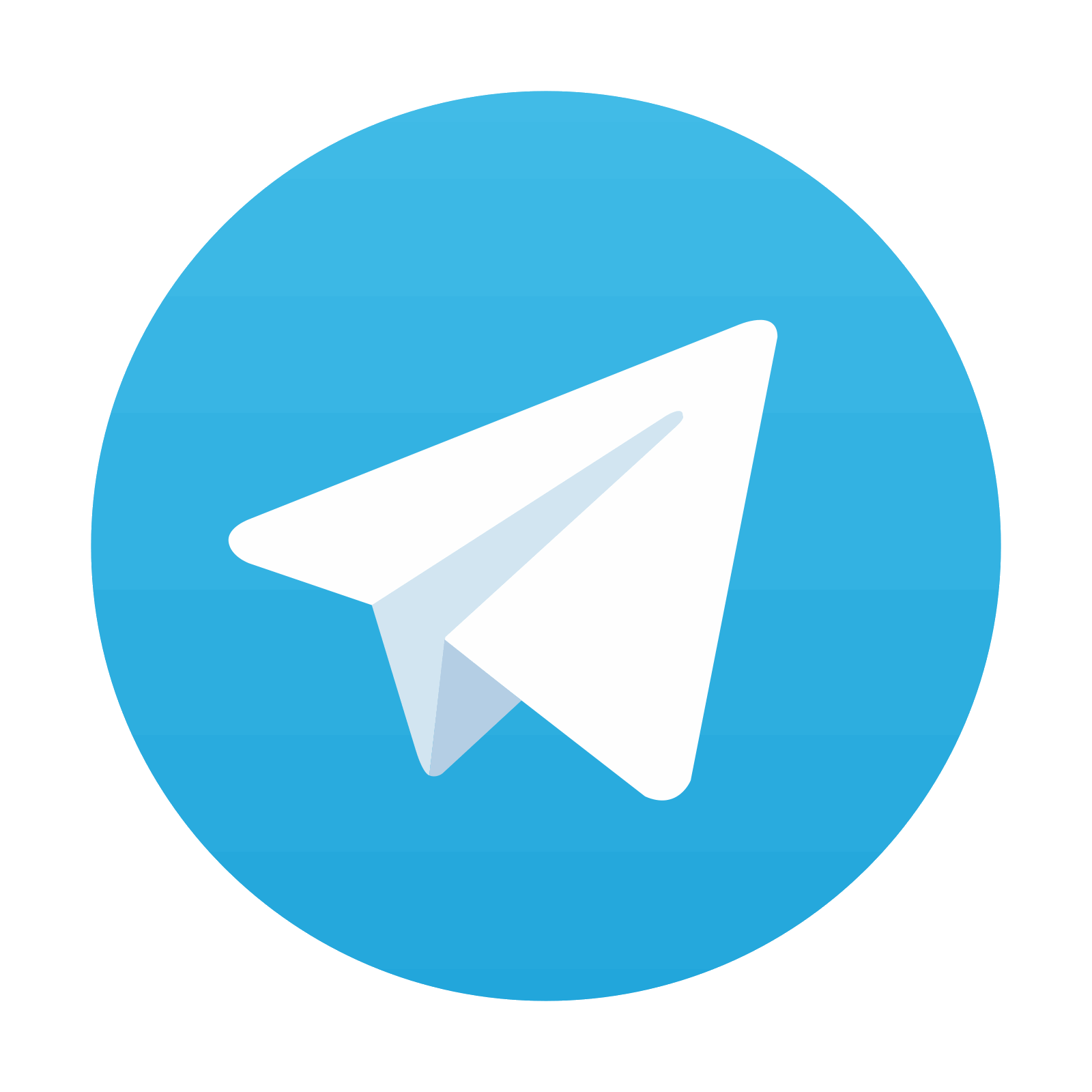
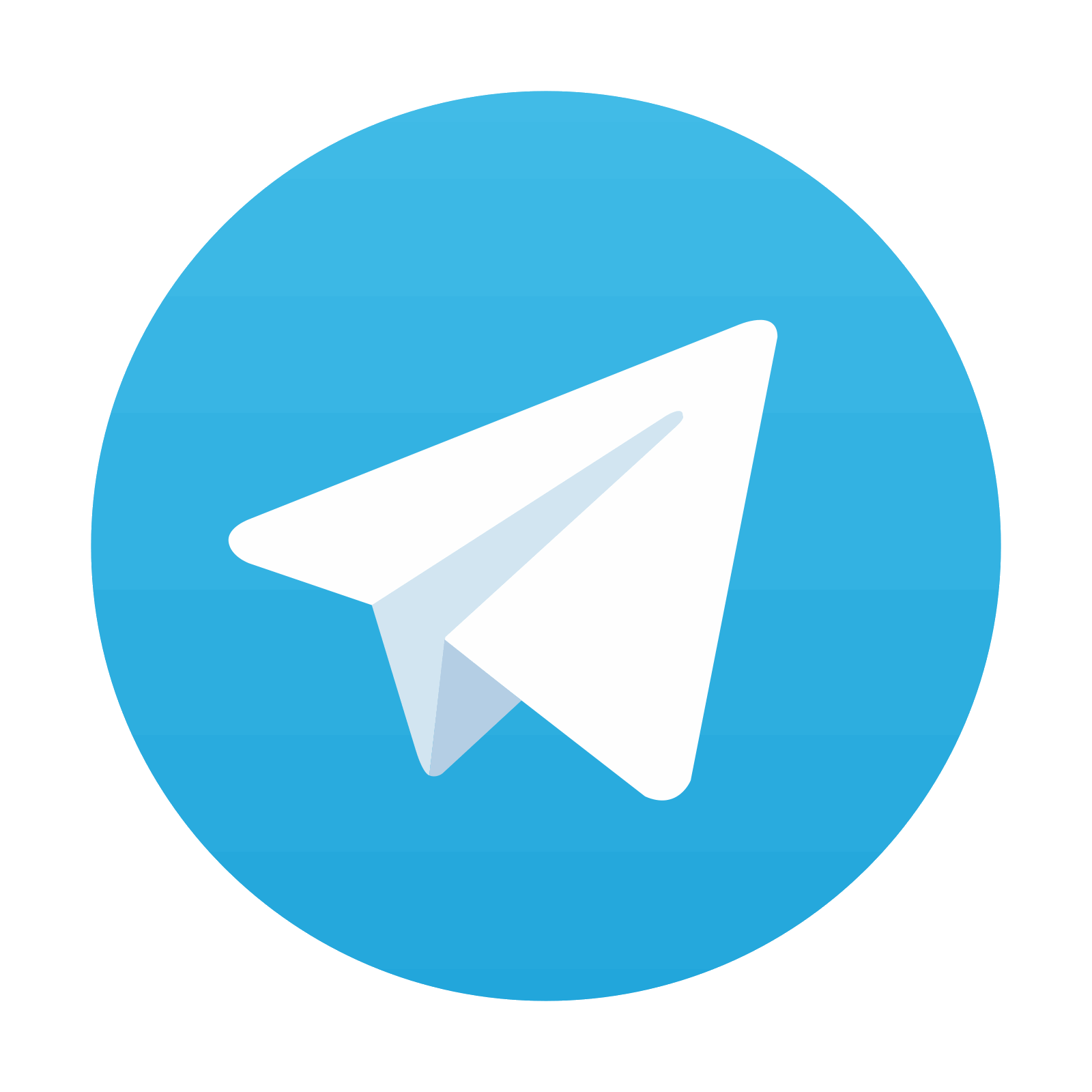
Stay updated, free articles. Join our Telegram channel
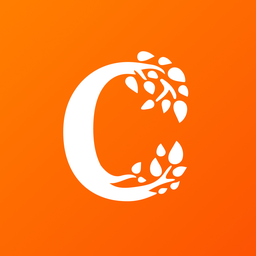
Full access? Get Clinical Tree
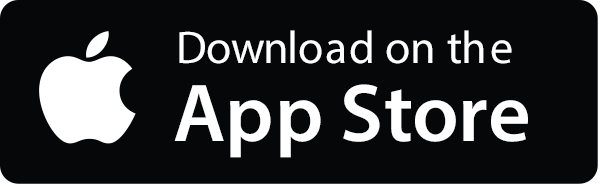
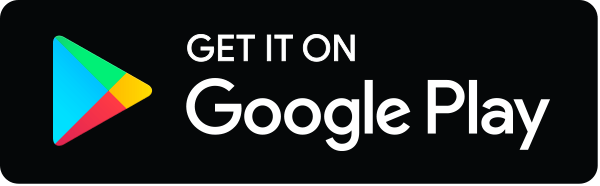
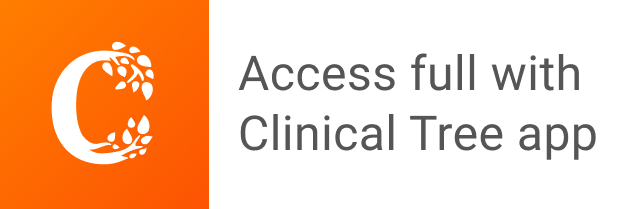