CHAPTER 13 The lumbar spine
Spinal problems are among the most common conditions encountered in physical medicine. More working days are lost because of back pain than any other single condition, and sport does not escape this epidemic. Table 13.1 lays out the cost to the individual and to society as a whole.
Table 13.1 Low back pain: the scope of the problem
![]() |
GP—general practitioner; LBP—low back pain; NHS—National Health Service.Compiled from Fryomoyer and Cats-Baril (1991) Clinical Standards Advisory Group (1994), CSP (2004), Airaksinen et al. (2005).
In sport, the frequency of back pain suffering presents a similar challenge. Exercise itself has a positive effect on the low back both in terms of injury prevention and rehabilitation. Those with an activity level of at least 3 hours per week have a generally lower lifetime risk of low back pain (Harreby et al., 1997). After an injury has occurred, exercise therapy has been shown to be effective at returning patients to their daily activities and to work (Van Tulder et al., 2000; Mercer et al., 2006), and has been recommended as the mainstay of treatment for this region (Waddell, Feder and Lewis, 1997).
Although exercise is beneficial to the low back, the varied activities within sport subject the spine to significant stress which often results in injury. In terms of percentage, 10–20% of all sports injuries involve the spine (Thompson, 2002), but this percentage differs between sports (Table 13.2).
Table 13.2 Back pain in specific sports
Sport | Effect |
---|---|
Canoeing | 22.5% suffer from lumbago |
Cross-country skiing | 64% suffer from back pain |
Cycling | Incidence of back pain as high at 73.2% |
Golf | Lifetime incidence as high at 63% |
Gymnastics | 86% of rhythmic gymnasts report low back pain. 63% of Olympic female gymnasts have MRI abnormalities |
Rowing | Mechanical back pain most common type |
Squash | 51.8% of competitive players report back injury |
Swimming | 37% suffer back pain especially with breaststroke and butterfly |
Triathlon | 32% suffer low back pain |
Windsurfing | Low back pain most common ailment |
Yachting | Lumbosacral sprain most common injury (29%) |
From Thompson, B. (2002) How should athletes with chronic low back pain be managed in primary care? In Evidence Based Sports Medicine (eds D. MacAuley and T. Best). BMJ Books, London. With permission.
A detailed study of back pain is outside the scope of this book, but it is necessary to look at a number of features of spinal injury which are important within the context of sport. Much of the material for the initial parts of this section is modified from Norris (1995), and the reader is referred to that article series and Norris (2008) for a more in-depth review.
Structure
The spinal disc
The annulus is composed of layers of fibrous tissue arranged in concentric bands (Fig. 13.1). Each band has fibres arranged in parallel, and the various bands are in turn angled at 45° to each other. The bands are more closely packed anteriorly and posteriorly than they are laterally, and those innermost are the thinnest. Each disc has about 20 bands in all, and fibre orientation, although partially determined at birth, is influenced by torsional stresses in the adult (Palastanga, Field and Soames, 1989). The posterolateral regions have a more irregular make-up, and this may be one reason why they become weaker with age, predisposing them to injury.
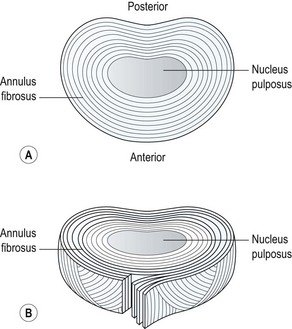
Figure 13.1 (A) Concentric band of annular fibres. (B) Horizontal section through a disc.
From Oliver and Middleditch (1991) with permission.
The annular fibres pass over the edge of the cartilage end plate of the disc, and are anchored to the bony rim of the vertebra and to its periosteum and body. The attaching fibres are actually interwoven with the fibres of the bony trabeculae of the vertebral body. The outer layer of fibres blend with the posterior longitudinal ligament, but the anterior longitudinal ligament has no such attachment (Vernon-Roberts, 1987).
The nucleus pulposus is a soft hydrophilic (water attracting) substance taking up about 25% of the total disc area. It is continuous with the annulus, but the nuclear fibres are far less dense. The spaces between the collagen fibres are filled with proteoglycan, giving the nucleus its water-retaining capacity, and making it a mechanically plastic material. The area between the nucleus and annulus is metabolically very active and sensitive to physical force and chemical and hormonal influence (Palastanga, Field and Soames, 1989). The proteoglycan content of the nucleus decreases with age, but the collagen volume remains unchanged. As a consequence, the water content of the nucleus reduces. In early life the water content may be as high as 80–90%, but this decreases to about 70% by middle age.
The lumbar discs are the largest avascular structures in the body. The nucleus itself is dependent upon fluid exchange by passive diffusion from the margins of the vertebral body and across the cartilage end plate. Diffusion takes place particularly across the centre of the cartilage end plate which is more permeable than the periphery. There is intense anaerobic activity within the nucleus (Holm et al., 1981), which could lead to lactate build up and a low oxygen tension, placing the nuclear cells at risk. Inadequate adenosine triphosphate (ATP) supplies could lead to cell death.
The facet joint
The facet (zygapophyseal) joints are synovial joints formed between the inferior articular process of one vertebra and the superior articular process of its neighbour. As with all typical synovial joints, they have articular cartilage, a synovial membrane and a joint capsule. However, the zygapophyseal joints do have a number of unique features (Bogduk and Twomey, 1991).
The capsule is a lax structure which enables the joint to hold about 2 ml of fluid. It is replaced anteriorly by the ligamentum flavum, and posteriorly it is reinforced by the deep fibres of multifidus. The joint leaves a small gap at its superior and inferior poles creating the subscapular pockets (Fig. 13.2). These are filled with fat, contained within the synovial membrane. Within the subscapular pocket lies a small foramen for passage of the fat in and out of the joint as the spine moves.
Intracapsularly there are three structures of interest (Fig. 13.3). The first is the connective tissue rim, a thickened wedge-shaped area which makes up for the curved shape of the articular cartilage in much the same way as the menisci of the knee do. The second structure is an adipose tissue pad, a 2 mm fold of synovium filled with fat and blood vessels. The third structure is the fibroadipose meniscoid, a 5 mm leaf-like fold which projects from the inner surfaces of the superior and inferior capsules. These latter two structures have a protective function. During flexion, the movement of the articular facets leaves some of their cartilage exposed. Both the adipose tissue pad and the fibroadipose meniscoid are able to cover these exposed regions (Bogduk and Engel, 1984).
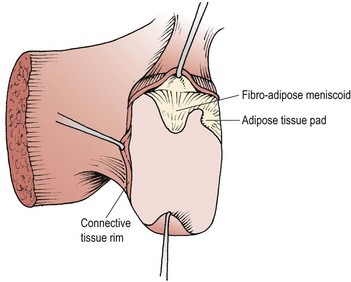
Figure 13.3 Intra-articular structures of the lumbar zygapophyseal joints.
From Bogduk and Twomey (1991), with permission.
With ageing, the cartilage of the zygapophyseal joint can split parallel to the joint surface, pulling a portion of joint capsule with it. The split of cartilage with its attached piece of capsule forms a false intra-articular meniscoid (Taylor and Twomey, 1986). Normally, the fibroadipose meniscus itself is drawn out from the joint on flexion, and should move back in with extension. However, if the meniscoid fails to move back, it will buckle and remain under the capsule, causing pain and acute locking (Bogduk and Jull, 1985). A mobilization or manipulation which combines flexion and rotation may allow the meniscoid to reduce and so relieve pain.
The facet has an overlapping neural supply, with ascending, local and descending facet branches coming from the posterior primary ramus. The nerve endings in the facet joint capsules are similar to those in the annulus of the disc, and although the disc is more sensitive, the facet joints can be a source of referred pain to the lower limb (Hirsch, Ingelmark and Miller, 1963), but not neurological deficit (Mooney and Robertson, 1976).
Spinal loading
Vertebral body
Within the vertebra itself, compressive force is transmitted by both the cancellous bone of the vertebral body and the cortical bone shell. Up to the age of 40, the cancellous bone contributes between 25% and 55% of the strength of the vertebra. After this age the cortical bone shell carries a greater proportion of load as the strength and stiffness of the cancellous bone reduces with decreasing bone density due to ageing (Rockoff, Sweet and Bleustein, 1969). As the vertebral body is compressed, blood flows from it into the subchondral post-capillary venous network (Crock and Yoshizawa, 1976). This process reduces the bone volume and dissipates energy (Roaf, 1960). The blood returns slowly as the force is reduced, leaving a latent period after the initial compression, during which the shock-absorbing properties of the bone will be less effective. Exercises which involve prolonged periods of repeated shock to the spine, such as jumping on a hard surface, are therefore more likely to damage the vertebrae than those which load the spine for short periods and allow recovery of the vertebral blood-flow before repeating a movement.
Intervertebral disc
Weight is transmitted between adjacent vertebrae by the lumbar intervertebral disc. The annulus fibrosis of a disc, when healthy, has a certain bulk and will resist buckling. When loads are applied briefly to the spine, even if the nucleus pulposus of a disc has been removed, the annulus alone exhibits a similar load-bearing capacity to that of the fully intact disc (Markolf and Morris, 1974). When exposed to prolonged loading, however, the collagen lamellae of the annulus will eventually buckle.
The application of an axial load will compress the fluid nucleus of the disc causing it to expand laterally. This lateral expansion stretches the annular fibres, preventing them from buckling. A 100 kg axial load has been shown to compress the disc by 1.4 mm and cause a lateral expansion of 0.75 mm (Hirsch and Nachemson, 1954). The stretch in the annular fibres will store energy which is released when the compression stress is removed. The stored energy gives the disc a certain springiness which helps to offset any deformation which occurred in the nucleus. A force applied rapidly will not be lessened by this mechanism, but its rate of application will be slowed, giving the spinal tissues time to adapt.
Deformation of the disc occurs more rapidly at the onset of axial load application. Within 10 minutes of applying an axial load the disc may deform by 1.5 mm. Following this, deformation slows to a rate of 1 mm per hour (Markolf and Morris, 1974), accounting for a subject’s loss of height throughout the day. Reduction in disc height slackens the collagen fibres in both the annulus and the spinal ligaments. A 2-hour compressive force which reduces the disc height by 1.1 mm has been shown to reduce resistance to flexion by 41% and increase flexion range by 12% (Adams et al., 2002). The range of flexion increases gradually throughout the day as tissues slacken and resistance is reduced. The greatest increase is seen in the first hours of rising.
Under constant loading the discs exhibit creep, meaning that they continue to deform even though the load they are exposed to is not increasing. Compression causes a pressure rise, leading to fluid loss from both the nucleus and annulus. About 10% of the water within the disc can be squeezed out by this method (Kraemer, Kolditz and Gowin, 1985), the exact amount being dependent on the size of the applied force and the duration of its application. The fluid is absorbed back through pores in the cartilage end plates of the vertebra when the compressive force is reduced.
Exercises which axially load the spine have been shown to result in a reduction in subject height through discal compression. Compression loads of 6–10 times bodyweight have been shown to occur in the L3–L4 segment during a squat exercise in weight training for example (Cappozzo et al., 1985). Average height losses of 5.4 mm over a 25-minute period of general weight training, and 3.25 mm after a 6 km run have also been shown (Leatt, Reilly and Troup, 1986). Static axial loading of the spine with a 40 kg barbell over a 20-minute period can reduce subject height by as much as 11.2 mm (Tyrrell, Reilly and Troup, 1985). Clearly, exercises which involve this degree of spinal loading are unsuitable for individuals with discal pathology (Table 13.3).
Table 13.3 Effect of exercise on the spinal disc
The vertebral end plates of the discs are compressed centrally, and are able to undergo less deformation than either the annulus or the cancellous bone. The end plates are, therefore, likely to fail (fracture) under high compression (Norkin and Levangie, 1992). Discs subjected to very high compressive loads show permanent deformation but not herniation (Farfan et al., 1970; Markolf and Morris, 1974). However, such compression forces may lead to herniation of nuclear material through the disc endplate known as a Schmorl’s node (Bernhardt et al., 1992) (Fig. 13.4 Adams et al., 2002).
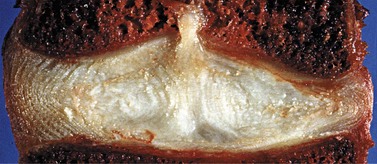
Figure 13.4 Schmorl’s node: herniation of nuclear material through the disc endplate.
Reproduced from Adams et al. (2002).
Bending and torsional stresses on the spine, when combined with compression, are more damaging than compression alone, and degenerated discs are particularly at risk. Average failure torques for normal discs are 25% higher than for degenerative discs (Farfan et al., 1970). Degenerative discs also demonstrate poorer viscoelastic properties and therefore a reduced ability to attenuate shock.
Age related changes
We have seen that with age the back wall of the disc weakens, the end plates become brittle and the disk reduces its water content from 90% in childhood to 70% in middle age. In addition, the disc’s reaction to a compressive stress changes with age, because the ability of the nucleus to transmit load relies on its high water content. The hydrophilic nature of the nucleus is the result of the proteoglycan it contains, and as this changes from about 65% in early life to 30% by middle age (Bogduk and Twomey, 1987), the nuclear load-bearing capacity of the disc reduces. When the proteoglycan content of the disc is high, up to the age of 30 years in most subjects, the nucleus pulposus acts as a gelatinous mass, producing a uniform fluid pressure. After this age, the lower water content of the disc means that the nucleus is unable to build as much fluid pressure. As a result, less central pressure is produced and the load is distributed more peripherally, eventually causing the annular fibres to become fibrillated and to crack (Hirsch and Schajowicz, 1952).
Brown pigmentation is seen which is an indication of change in the collagenous tissue (Adams et al., 2002), and the nucleus becomes dry and fibrous. As the disc drys, the annulus takes more of the compressive strain of weight bearing. However, the annulus itself weakens through the accumulation of defects and fissures over time (Fig. 13.5).
Movements of the spine
Discal pressure changes
If the pressure at the L3 disc for a 70 kg standing subject is said to be 100%, supine lying reduces this pressure to 25%. The pressure variations increase dramatically as soon as the lumbar spine is flexed and tissue tension increases (Fig. 13.6). The sitting posture increases intradiscal pressure to 140%, while sitting and leaning forward with a 10 kg weight in each hand increases pressure to 275% (Nachemson, 1987). The selection of an appropriate starting position for trunk exercise is therefore of great importance. Superimposing spinal movements from a slumped sitting posture, for example, would place considerably more stress on the spinal discs than the same movement beginning from crook lying.
As the lumbar spine flexes, the lordosis flattens and then reverses at its upper levels. Reversal of lordosis does not occur at L5–S1 (Pearcy, Portek and Shepherd, 1984). Flexion of the lumbar spine involves a combination of anterior sagittal rotation and anterior translation. As sagittal rotation occurs, the articular facets move apart, permitting the translation movement to occur. Translation is limited by impaction of the inferior facet of one vertebra on the superior facet of the vertebra below (Fig. 13.7). As flexion increases, or if the spine is angled forward on the hip, the surface of the vertebral body will face more vertically increasing the shearing force due to gravity. The forces involved in facet impaction will therefore increase to limit translation of the vertebra and stabilize the lumbar spine. Because the zygapophyseal joint has a curved articular facet, the load will not be concentrated evenly across the whole surface, but will be focused on the anteromedial portion of the facets.
The sagittal rotation movement of the zygapophyseal joint causes the joint to open and is therefore limited by the stretch of the joint capsule. Additionally, the posteriorly placed spinal ligaments will also be tightened. Analysis of the contribution to limitation of sagittal rotation within the lumbar spine, through mathematical modelling, has shown that the disc limits movement by 29%, the supraspinous and interspinous ligaments by 19% and the zygapophyseal joint capsules by 39% (Adams, Hutton and Stott, 1980).
Extension
During extension the anterior structures are under tension while the posterior structures are first unloaded and then compressed, depending on the range of motion. With extension movements the vertebral bodies will be subjected to posterior sagittal rotation. The inferior articular processes move downwards causing them to impact against the lamina of the vertebra below. Once the bony block has occurred, if further load is applied the upper vertebra will axially rotate by pivoting on the impacted inferior articular process. The inferior articular process will move backwards, overstretching, and possibly damaging, the joint capsule (Yang and King, 1984). With repeated movements of this type, eventual erosion of the laminal periosteum may occur (Oliver and Middleditch, 1991). At the site of impaction, the joint capsule may catch between the opposing bones giving another cause of pain (Adams and Hutton, 1983). Structural abnormalities can alter the axis or rotation of the vertebra, so considerable variation between subjects exists (Klein and Hukins, 1983).
Rotation
During rotation, torsional stiffness is provided by the outer layers of the annulus, by the orientation of the zygapophyseal joints, and by the cortical bone shell of the vertebral bodies themselves. In rotation movements, the annular fibres of the disc will be stretched according to their direction. As the two alternating sets of fibres are angled obliquely to each other, some of the fibres will be stretched while others relax. A maximum range of 3° of rotation can occur before the annular fibres will be microscopically damaged, and a maximum of 12° before tissue failure (Bogduk and Twomey, 1987). As rotation occurs, the spinous processes separate, stretching the supraspinous and interspinous ligaments. Impaction occurs between the opposing articular facets on one side causing the articular cartilage to compress by 0.5 mm for each 1° of rotation, providing a substantial buffer mechanism (Bogduk and Twomey, 1987). If rotation continues beyond this point, the vertebra pivots around the impacted zygapophyseal joint causing posterior and lateral movement (Fig. 13.8). The combination of movements and forces which occur will stress the impacted zygapophyseal joint by compression, the spinal disc by torsion and shear, and the capsule of the opposite zygapophyseal joint by traction. The disc provides only 35% of the total resistance (Farfan et al., 1970).
Lateral flexion
When the lumbar spine is laterally flexed, the annular fibres towards the concavity of the curve are compressed and will bulge, while those on the convexity of the curve will be stretched. The contralateral fibres of the outer annulus and the contralateral intertransverse ligaments help to resist extremes of motion (Norkin and Levangie, 1992). Lateral flexion and rotation occur as coupled movements. Rotation of the upper four lumbar segments is accompanied by lateral flexion to the opposite site. Rotation of the L5–S1 joint occurs with lateral flexion to the same side.
End-range spinal stress in sport
In addition, end-range stress can be experienced with postural changes and an alteration in the control of movement within the lumbar spine. Clinically, a number of directional patterns have been described (Sullivan: O’, 2000). Flexion of the lumbar spine is seen with a gross reduction in the depth of the lumbar lordosis (Fig. 13.9). The athlete suffers pain when semi-flexed postures are maintained, and with prolonged sitting activities. When put in a four point kneeling position, the lumbar spine remains flexed. Extension of the lumbar spine is seen in the lordotic posture with the pelvis anteriorly tilted. In standing, and especially during extension movements of the whole spine or hip, the lumbar spine appears to ‘hinge’ as a single level rather than extend through its whole length. Lateral flexion movements are seen with tightness to the lateral flexors (quadratus lumborum and lateral external oblique). This is brought to the fore with single leg standing activities. Here, the patient, instead of transferring bodyweight with the pelvis, laterally flexes the spine and a noticeable scoliosis is apparent.
Discal injury
During flexion, extension and lateral flexion, one side of the disc is compressed and the other stretched. In flexion, the axis of motion passes through the nucleus, but with extension the axis moves forwards (Klein and Hukins, 1981). This fact, coupled with the increased range of motion during flexion, makes it the more dangerous movement. Combinations of torsion and flexion place the disc at particular risk from plastic deformation, which stretches the annular fibres irreversibly, and may cause fibre damage.
When hyperflexion takes place, the supraspinous and interspinous ligaments will overstretch, reducing the support to the lumbar spine. Circumferential tearing will occur to the disc annulus posterolaterally, usually at the junction between the disc lamina and end plate (Oliver and Middleditch, 1991). The outer annular fibres are innervated, a possible cause of the ‘dull ache’ in the lumbar spine which often precedes disc prolapse. Rotation strain will increase the likelihood of these injuries. Although rotation is limited in the lumbar spine, it is increased significantly as a result of facet joint degeneration and during flexion as the facets are separated.
Posterolateral radial fissuring occurs later, and connects the disc nucleus to the circumferential tear, allowing the passage of nuclear material towards the outer edge of the disc. This type of injury has been produced experimentally during discal compression in a combined flexed and laterally flexed posture (Adams and Hutton, 1982). An annular protrusion can occur when the pressure of the displaced nuclear material causes the annulus to bulge. Eventually, nuclear material is extruded (herniated) through the ruptured annular wall (Fig. 13.10).
Radiographic investigations of discal movement have been made by inserting metal pins into the lumbar nucleus pulposus and asymmetrically loading the disc (reported in McKenzie, 1990). These have shown that the disc migrates towards the area of least load. When the asymmetrical load was removed, the nucleus remained displaced, but its relocation was accelerated by compression in the opposite direction or by traction.
A number of studies have investigated the phenomenon of discal nuclear movement within the lumbar discs. Beattie, Brooks and Rothstein (1994) showed movement during extension with healthy discs but not with degenerative discs, and Edmondston, Song and Bricknell (2000) demonstrated 6.7% anterior displacement between flexion and extension in L1/2, L2/3 and L5/S1. Fennell, Jones and Hukins (1996) used MRI scanning to demonstrate anterior movement during extension.
Spinal ligaments
A number of spinal ligaments are of concern to the biomechanics of the lumbar spinal segment: the anterior and posterior longitudinal ligaments, the intertransverse ligament, the ligamentum flavum, the interspinous and supraspinous ligaments and the capsular ligaments of the facet joint (Fig. 13.11). A general reduction in energy absorption of all the ligaments has been found with age (Tkaczuk, 1968). The stiffest is the posterior longitudinal ligament and the most flexible the supraspinous (Panjabi, Jorneus and Greenstein, 1984). The ligamentum flavum in the lumbar spine is pre-tensioned (resting tension) when the spine is in its neutral position, a situation which compresses the disc. This ligament has the highest percentage of elastic fibres of any tissue in the body (Nachemson and Evans, 1968), and contains nearly twice as much elastin as collagen. With age and degeneration there is a reduction of the elastin content of the the ligamentum flavum, and calcification is sometimes apparent (Adams et al., 2002). The anterior longitudinal ligament and joint capsules have been found to be the strongest, while the interspinous and posterior longitudinal ligaments are the weakest (Panjabi, Hult and White, 1987).
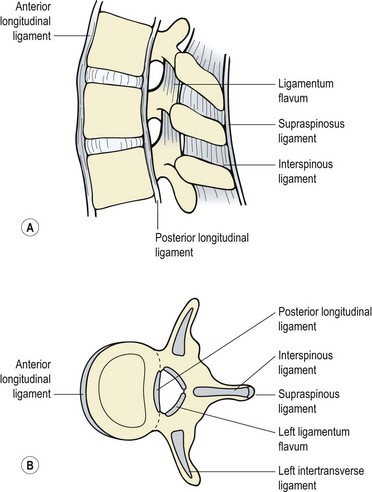
Figure 13.11 Ligaments of the spinal segment. (A) Side view. (B) Superior view.
Reprinted by permission from Norris, C.M. (2000) Back Stability. Human Kinetics, Champaign, Illinois.
The ligaments act rather like rubber bands, resisting tensile forces but buckling under compressive loads (Fig. 13.12). They must allow adequate motion and fixed postures between vertebrae, enabling a minimum amount of muscle energy to be used. In addition, they protect the spine by restricting motion and in particular protect the spinal cord in traumatic situations, where high loads are applied at rapid speeds. In this situation, the ligaments absorb large amounts of energy.
When loaded repeatedly, they become stiffer, and the hysteresis is less marked, making the longitudinal ligaments more prone to fatigue failure (Hukins, 1987). The supraspinous and interspinous ligaments are further from the flexion axis, and therefore need to stretch more than the posterior longitudinal ligament when they resist flexion.
Low back pain
The exact structure which is affected in low back pain is open to discussion, and often it is virtually impossible to identify precisely which tissue is causing a patient’s symptoms (Spitzer, LeBlanc and Dupuis, 1987). Diagnostic labels may often be misleading, with pathology identified which does not necessarily relate to the patient’s symptoms. Identification of the movement dysfunction or movement impairment (Sahrmann, 2002) may be a better guide to effective rehabilitation.
To this end, the approach taken by McKenzie (1981) is extremely useful. Back pain may be classified as mechanical or chemical (non-mechanical) in origin. Mechanical pain is produced by deformation of structures containing nociceptive nerve endings, and there is a clear correlation between certain body positions and the patient’s symptoms.
Examination of the back
Screening examination
Examination of the lumbar spine can be either very complex or relatively simple, depending on the approach taken. The reliability and reproducibility of tests for the spine increases when the information to be gained from the tests is kept to a minimum (Nelson et al., 1979). For this reason, the work of Cyriax (1982) and McKenzie (1981) is valuable as it provides enough information to treat the majority of patients. In addition, the tests tell the practitioner when further investigation is necessary.
Diagnostic triage
Diagnostic triage extends the screening examination by categorizing low back pain into three types: simple backache, nerve root pain and serious pathology (Table 13.4).
After Waddell, G., Feder, G. and Lewis, M. (1997) Systematic reviews of bed rest and advice to stay active for acute low back pain. British Journal of General Practice, 47, 647–652, and Norris (2000).
Where examination reveals non-mechanical pain in a young (under 20) or older (over 55) athlete, specialist investigation is required. This is especially the case where there is a previous history of an associated medical condition, or if the patient has been unwell, shows an obvious structural deformity of the spine or demonstrates gait disturbance. Where altered sensation is present in the ‘saddle’ area (perineum and genitals) further investigation is required as this indicates possible disc protrusion of the lower sacral nerve roots. Where this is present with difficulty in passing urine, an inability to retain urine and/or a lack of sensation when the bowels are opened, there is a possibility of compression of the cauda equina and immediate emergency referral is required (Kesson and Atkins, 1998; Magee, 2002).
The straight leg raise
The straight leg raise (SLR) or Lasegue’s sign is a widely used test to assess the sciatic nerve in cases of back pain. Although widely used, the test has limited diagnostic accuracy when diagnosing herniated discs. In a systematic review of 11 studies assessing the accuracy of SLR against surgery as a reference standard, Deville et al. (2000) found a low specificity of 0.26 and a sensitivity of 0.91.
In addition to its effect on the sciatic nerve, the SLR test also places stretch on the hamstrings, buttock tissues, sacroiliac joint, posterior lumbar ligaments and facet joints in addition to lengthening the spinal canal (Urban, 1986). Confirmation that the nerve root is the source of pain may be improved by raising the leg to the point of pain and then lowering it a few degrees. The neuromeningeal structures are then further stretched either from below by dorsiflexing the foot, or applying firm pressure to the popliteal fossa over the posterior tibial nerve. Pressure from above is produced by flexing the cervical spine. When performing the SLR, as the leg is raised the knee should not be allowed to bend and the pelvis should stay on the couch.
The dura within the spinal canal is firmly attached to the foramen magnum above and the filum terminale below. Trunk flexion causes the spinal canal to lengthen and therefore stretches the dura, whereas extension, by shortening the canal, induces dural relaxation allowing the sheath to fold. The neuromeningeal pathway is elastic, so tension imparted at one point will spread throughout the whole length of the spine. As the SLR is performed, the initial motion is of the nerve at the greater sciatic notch. As hip flexion goes through 35°, movement occurs proximal to the ala of the sacrum, and during the next 35° the movement is at the intervertebral foramen itself. The last degrees of the SLR do not produce further nerve movement, but simply increase the tension over the whole course of the nerve (Grieve, 1970) (Fig. 13.13).
Testing the unaffected leg (crossed SLR or ‘well leg’ test) may also give symptoms. This manoeuvre pulls the nerve root and dura distally and medially, but increases the pressure on the nerve complex by less than half that of the standard SLR test. When the ipsilateral SLR causes pain, it simply means that one of the tissues connected to the nerve pathway is sensitized. Because the crossed SLR stretches the neural structures less, the resting tension of these tissues must be higher to cause pain. The crossed SLR may therefore be a more reliable predictor of large disc protrusions than the ipsilateral SLR (Urban, 1986).
Slump test
The slump test is used to assess tension in the pain-sensitive structures around the vertebral canal or intervertebral foramen, and to ensure that these structures are able to stretch properly (Maitland, 1986; Butler, 1991). To perform the manoeuvre the patient sits unsupported over the couch side with the knees together and flexed to 90°. The posterior thigh is in contact with the couch. The patient is then instructed to relax the spine completely and ‘slump’ forward, keeping the cervical spine in its neutral position (‘look forwards, not down’). The therapist (standing at the side of the patient) places overpressure onto the patient’s shoulders to increase the movement, attempting to bow the spine rather than increase hip flexion (Fig. 13.14A).
From this position the patient is asked to flex the neck (‘chin to chest’) and then straighten the leg on the unaffected side first (Fig. 13.14B). In each case the examiner places overpressure on the area and assesses the result. The athlete is then asked to dorsiflex the ankle (‘pull your toes up’). Neck flexion is slowly released, and the response monitored. The opposite leg is then tested. A normal test result is one where there is a pain-free lack of knee extension by about 30° and slight central pain over T9/10 (Maitland, 1986).
Mechanical therapy
Three mechanical conditions are recognized in the lower back: the postural syndrome, dysfunction and derangement (McKenzie, 1981).
Postural syndrome
The most important part of management with the postural syndrome is patient education. To this end, the slouch-overcorrect procedure for correcting sitting posture is useful (McKenzie, 1981). The patient sits on a stool, and is allowed to slouch into an incorrect sitting posture for some time until back pain ensues. He or she is then taught a position of maximum lordosis, and learns how to change rapidly and at will, from the incorrect slouch to this overcorrect maximum lordosis. Once the patient has seen the relationship between poor sitting posture and pain, he or she is taught a correct sitting posture mid-way between the two extreme movement ranges. The use of a lumbar pad or roll is helpful to maintain the lordosis in sitting.
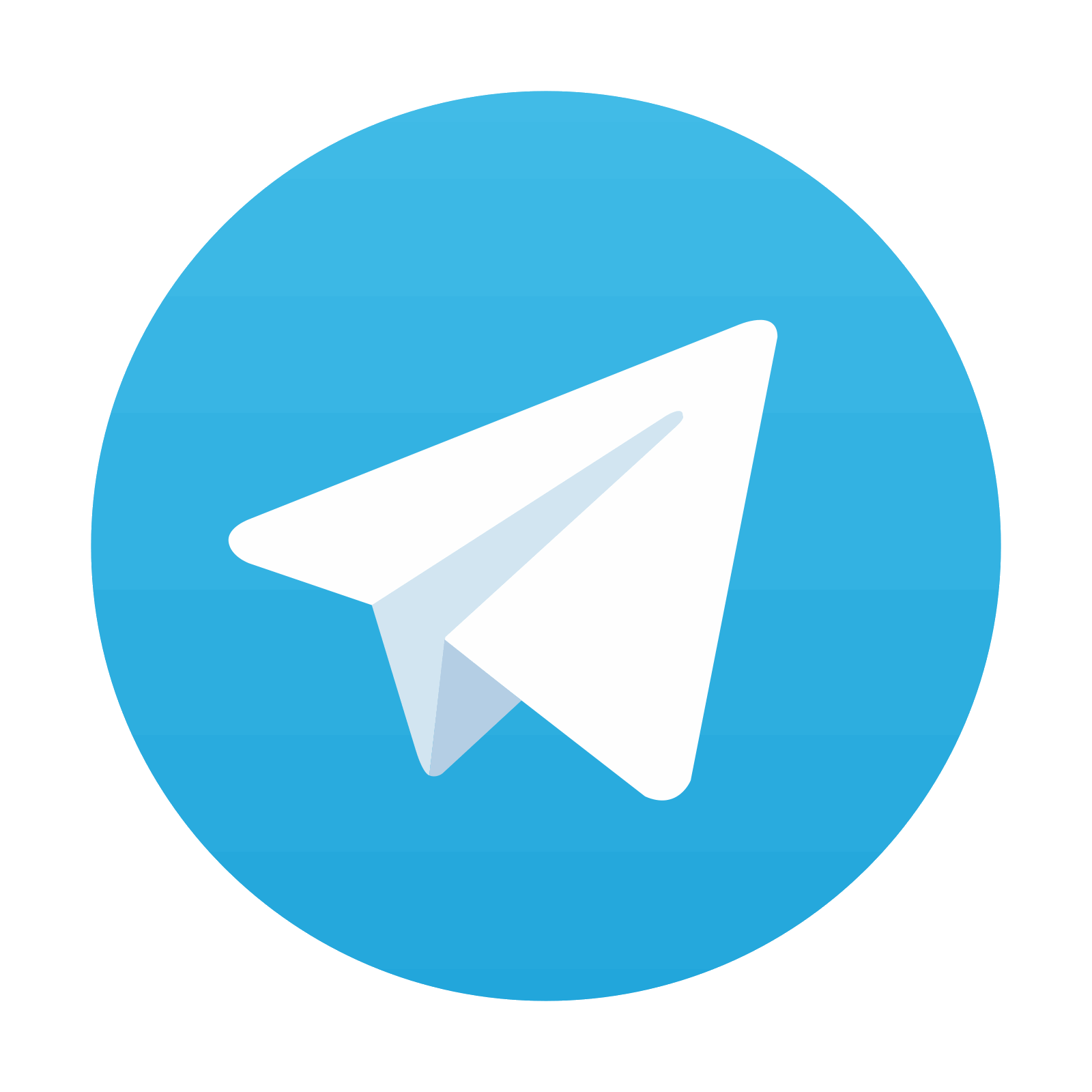
Stay updated, free articles. Join our Telegram channel
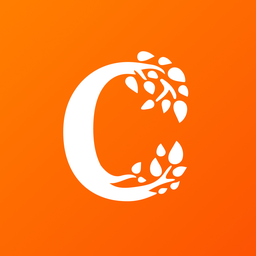
Full access? Get Clinical Tree
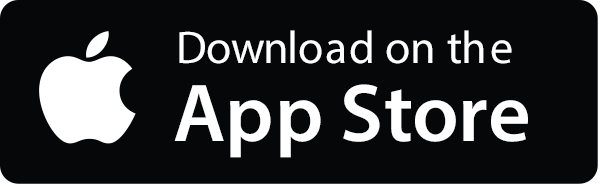
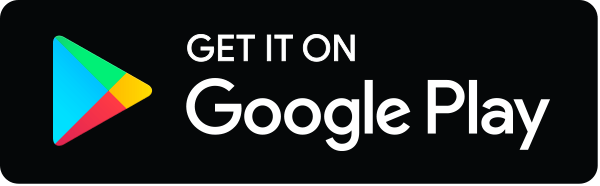