Fig. 23.1
Definition of a stem/progenitor cell. Criteria of stem/progenitor cell include clonogenicity, high proliferative capacity, self-renewal capability (from a single cell to reconstitute a tissue in which the cell was derived), and multipotency
23.2 Evidence of a Stem Cell System in the Intervertebral Disc
As mentioned above, investigation of the tissue-specific stem cell niche is a key factor in understanding the degenerative processes and tissue regeneration. Although research into the stem cell system in the intervertebral disc is still in its infancy, recent studies have shown evidence of a potential stem cell niche in the intervertebral disc region. Henriksson et al. (2009) attempted to detect cell proliferation zones and label-retaining cells with in vivo 5-bromo-2′-deoxyuridine (BrdU) labeling in rabbit discs. They also investigated the localization of progenitor cell markers (Notch1, Delta4, Jagged1, C-KIT, KI67, and Stro-1) with immunohistochemistry in the degenerated intervertebral discs of rabbits, rats, mini pigs, and humans. Although slow, ongoing proliferation was detectable in both the nucleus pulposus and annulus fibrosus regions. They also found a high number of BrdU-positive cells at the annulus fibrosus borders with the ligament zone and the perichondrium region at early time points, whereas only a few label-retaining cells were observed at later time points, identifying a stem cell niche-like pattern. This may support the findings of Melrose et al. (2007), who demonstrated spatial annular remodeling in experimentally injured ovine discs.
The recruitment of cells from the surrounding environment is another phenomenon often seen in the regenerative process. Using the BrdU in vivo labeling technique described above, Henriksson et al. (2011) observed BrdU-labeled cells in the intervertebral disc niche, adjacent to the epiphyseal plate at early time points, but at later time points, these cells were mainly in the outer region of the annulus fibrosus, suggesting possible migratory pathway. Tzaan and Chen (2011) have extended this concept by enhancing bone marrow cell migration to the disc by stimulation with granulocyte colony-stimulating factor. However, their results only demonstrated increased bone marrow cells in the endplate. Cell migration processes are thus underdefined and require substantial investigation.
There is increasing evidence that stem/progenitor cells are present in the intervertebral disc. Risbud et al. (2007) reported that cells isolated from degenerative human disc tissues expressed CD105, CD166, CD63, CD49a, CD90, CD73, p75 low-affinity nerve growth factor receptor, and CD133/1, proteins that are characteristic of MSC and represent the differentiation capacity of these cells toward osteogenesis, adipogenesis, and chondrogenesis. Feng et al. (2010) have demonstrated that the human degenerative annulus contains cells that express the MSC markers CD29, CD49e, CD51, CD73, CD90, CD105, CD166, CD184, and Stro-1 and two neuronal stem cell markers, nestin and neuron-specific enolase. In an in vitro assay, they differentiated annulus fibrosus-derived cells into adipocytes, osteoblasts, chondrocytes, neurons, and endothelial cells. Blanco et al. (2010) investigated MSC markers in nucleus pulposus cells isolated from degenerated discs and compared their differentiation capacities with those of bone marrow-derived MSC from the same patient. They found that nucleus pulposus-derived MSC fulfilled almost all the morphological, immunophenotypical, and differentiation criteria for MSC described by the International Society of Cell Therapy, except that nucleus-derived MSCs were unable to differentiate into adipocytes. Likewise, Liu et al. (2011) investigated the characteristics of MSC derived from degenerated human disc cartilage endplates and reported that the morphology, proliferation rate, cell cycle, immunophenotype, and expression of stem cell genes were similar to those isolated from the bone marrow. These research findings suggest that the stimulation of endogenous stem cell populations may be an effective strategy for treating intervertebral disc degeneration or to provide cells for the allogeneic transplantation of somatic-tissue-specific stem cells.
23.3 Commitment of Stem Cells Toward an Intervertebral Disclike Cell
In contrast to the small steps yet taken in investigating the endogenous stem cell system in the intervertebral disc, a number of studies have reported the utilization of MSC in new, targeted therapeutic strategies (Fig. 23.2). MSC utilization can be subdivided into three main types of studies. The first approach utilizes the multipotent differentiation capacity of MSC to induce stem cells to commit toward an intervertebral disclike cell. A considerable number of studies in the literature describe this strategy (Risbud et al. 2004; Li et al. 2005; Steck et al. 2005; Richardson et al. 2006; Sobajima et al. 2008; Vadalà et al. 2008; Wuertz et al. 2008; Chen et al. 2009; Kim et al. 2009; Le Maitre et al. 2009; Wei et al. 2009a; Korecki et al. 2010; Strassburg et al. 2010; Bertolo et al. 2012; Choi et al. 2011; Feng et al. 2011a; Luo et al. 2011; Purmessur et al. 2011; Ruan et al. 2012; Stoyanov et al. 2011). Various methods to induce MSC differentiation have been evaluated such as stimulation with growth factors under specific culture conditions, coculture with terminally differentiated intervertebral disc cells, or seeding cells onto a microenvironment-mimicking scaffold. Steck et al. (2005) compared the molecular phenotypes of human disc cells and articular chondrocytes to determine whether MSC can differentiate toward both cell types after transforming growth factor-β (TGFβ)-mediated induction in vitro. Their results demonstrated that the gene expression profile adopted by MSC resembled that of native disc tissue more closely than that of native joint cartilage. As well as TGFβ3, insulin-like growth factor 1 (IGF1), fibroblast growth factor 2, and platelet-derived growth factor BB have been shown to induce MSC differentiation toward nucleus pulposus-like cells (Ehlicke et al. 2010). Richardson et al. (2006) assessed the value of a coculture system with or without cell–cell contact to induce MSC differentiation toward a nucleus pulposus-like phenotype. Real-time quantitative polymerase chain reaction (RT-PCR) demonstrated a significant increase in the expression of nucleus pulposus marker genes in stem cells when the cells were cocultured with contact for 7 days, and this change was regulated by the cell ratio. However, no significant changes in marker gene expression was observed when the cells were cultured with either the nucleus pulposus cells or stem cells without contact, indicating a requirement for cell–cell contact in this induction process.
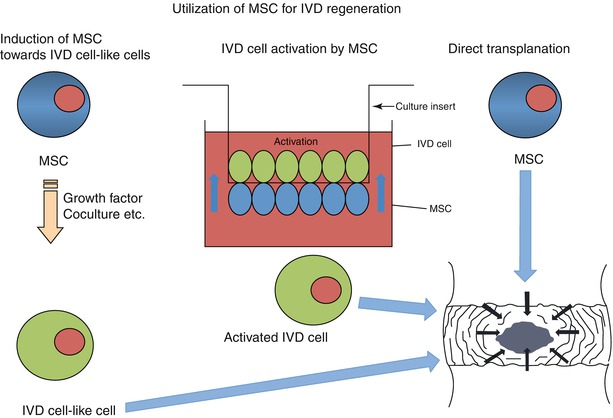
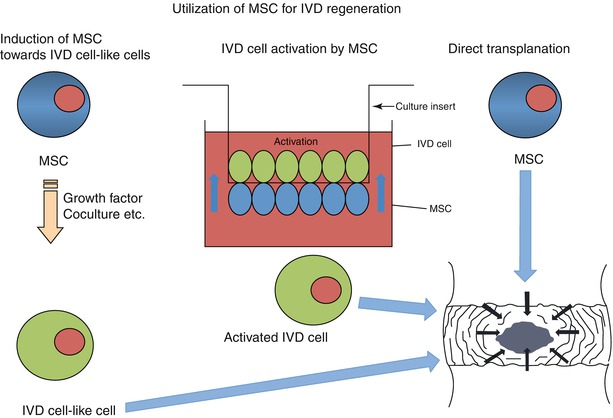
Fig. 23.2
Utilization of multipotent mesenchymal stromal cells for disc regeneration. Mesenchymal stem cells (MSCs) can be directly induced to differentiate toward an intervertebral disc cell (IVD) using growth factor and coculture techniques (left). In addition, activation can be achieved using coculture systems in which there is direct cell–cell contact (middle). Alternatively, MSC can be directly transplanted into the disc and commitment enhanced by the local conditions and the presence of resident host cells (right)
In a follow-up study, Strassburg et al. (2010) used human nucleus pulposus cells from degenerate and nondegenerate intervertebral discs to show that MSC differentiated into a nucleus pulposus-like phenotype following direct coculture with either of the tissues with significantly upregulated expression of the genes encoding SOX9, collagen VI, aggrecan, and versican, together with the simultaneous upregulation of growth differentiation factor 5 (GDF5), TGFβ1, IGF1, and connective tissue growth factor expression. The direct coculture of normal nucleus pulposus cells with MSC had no effect on the phenotype of the nucleus cells, whereas coculture with degenerated nucleus pulposus cells resulted in enhanced matrix gene expression in the degenerate cells, accompanied by increases in both TGFB1 and GDF5 gene expressions. These results suggest that cellular interactions between MSC and degenerated nucleus pulposus cells both stimulate MSC differentiation to a nucleus pulposus-like phenotype and promote the endogenous nucleus pulposus cell population to regain a nondegenerated phenotype, consequently enhancing matrix synthesis for self-repair. Similarly, when human nucleus pulposus cells were maintained in a pellet coculture with human MSC in different ratios, the 75:25 and 50:50 NP:MSC ratios yielded the greatest increases in extracellular matrix (ECM) production (Sobajima et al. 2008). Vadalà et al. (2008) also reported a reduction in the expression of collagen I and an increase in the expression of collagen II and aggrecan in MSCs after coculture with nucleus pulposus cells in alginate hydrogels, which allowed short-distance paracrine cell interactions. Stoyanov et al. (2011) have shown the important role of hypoxia, together with the addition of GDF5, in enhancing the expression of nucleus pulposus markers in a bone marrow-derived MSC coculture.
MSCs from sources other than the bone marrow have also been examined. Lu et al. (2007) studied the interactions between human nucleus pulposus cells and adipose-tissue-derived stem cells in Transwell coculture, using both monolayer and micromass configurations. A similar coculture study was performed by Chen et al. (2009) utilizing synovium-derived MSC. Vadalà et al. (2008) investigated muscle-derived MSC, and Ruan et al. (2012) assessed whether Wharton’s jelly cells could be induced to differentiate toward nucleus pulposus-like cells in similar coculture studies.
The effects of scaffold properties on the differentiation of MSC toward intervertebral disc cells have also been investigated. Bertolo et al. (2012) evaluated four types of matrices as scaffolds, approved as medical devices for other applications: two made of equine or porcine collagen, one of gelatin, and one of chitosan. They showed that although the collagen scaffolds induced better chondrogenic differentiation than the other scaffolds, the phenotype of the MSC was not fully equivalent to that of nucleus pulposus cells. Feng et al. (2011a) investigated the use of three-dimensional (3D) nanofibrous poly(l-lactide) scaffolds seeded with rabbit MSCs which were induced to differentiate along the nucleus pulposus pathway in a hypoxic chamber (2 % O2) in the presence of TGFβ1. The nanofibrous scaffold supported the differentiation of rabbit MSC toward a nucleus pulposus-like phenotype in vitro, with the upregulated expression of a few important nucleus-associated genes (encoding aggrecan, collagen II, and Sox-9), the abundant deposition of ECM (glycosaminoglycan and collagen II), and the continuous expression of the nucleus pulposus-specific marker, hypoxia-inducible factor 1-α (HIF1-α).
The effect of physiological stimulation on MSC differentiation toward intervertebral disclike cells has also been studied. Luo et al. (2011) cultured MSC under simulated microgravity in a chemically defined medium supplemented with TGFβ1 (positive control group). The results showed that MSC independently and spontaneously differentiated toward a nucleus pulposus-like phenotype under simulated microgravity without the addition of any external bioactive stimulant, such as factors from the TGFβ family, which were previously considered necessary.
Recently, another unique approach to inducing MSC was reported by Korecki et al. (2010). Because notochord-derived cells play a major role in nucleus development, they tested whether the culture medium from porcine notochordal cells could direct the differentiation of MSCs. Human MSC pellets were cultured first in serum-free medium for 4 days and then in notochordal cell-conditioned medium for 7 days. It was found that there was glycosaminoglycan accumulation and increased gene expression toward a nucleus pulposus-like phenotype, together suggesting that there was differentiation toward that of the intervertebral disc phenotype.
The results of these studies suggest that MSC from any source can be forced to express some of the molecular markers of intervertebral disc cells in vitro and many external stimuli can accelerate differentiation. However, at the end of the day, they do not develop beyond nucleus pulposus-like cells because the default differentiation pathway is determined by the localization of the MSC. The most important factor in studying the induction of MSC toward intervertebral disclike cells is to demonstrate their functional capacity in vivo.
23.4 Stem Cell Promotion of Disc Cell Differentiation
Another approach to the utilization of stem cells is to exploit their capacity to nourish other cells. Stem cells can act as feeder cells to stimulate target cells directly through cell–cell contact or indirectly through the secretion of various factors. In a rabbit disc cell culture, Yamamoto et al. (2004) showed that direct cell–cell contact between nucleus pulposus cells and MSC occurred across a membrane with 0.45 mm pores, which allowed only the cell processes to adhere to each other, without any more extensive contact between the cultured cells. Compared with the culture systems with no cell–cell contact, a coculture system that allowed intercellular adhesion between nucleus pulposus cells and MSC yielded a marked increase in target cell proliferation, DNA synthesis, and proteoglycan synthesis. This is possibly attributable to the increased secretion of cytokines found in the culture system.
An interesting study by He and Pei (2012) tested the effects of the ECM deposited by synovium-derived MSC on the rejuvenation of nucleus pulposus cells. The nucleus pulposus cells that were expanded on ECM grew much faster, were smaller, and had a more fibroblastic shape than those expanded in plastic flasks. ECM-treated nucleus pulposus cells acquired enhanced CD90 expression and higher mRNA levels of collagen I, collagen II, and collagen X and aggrecan as well as a robust redifferentiation capacity for up to six passages. The authors concluded that the ECM provides a tissue-specific microenvironment for the rejuvenation of nucleus pulposus cells with a higher proliferation rate and greater redifferentiation capacity. These characteristics may improve any future autologous disc cell-based minimally invasive therapeutic approach to the physiological reconstruction of a biologically functional disc in the clinical setting.
Another use of MSC is in the delivery of bioactive factors to resident disc cells. Meyerrose et al. (2010) suggested the use of MSC for the sustained in vivo production of supraphysiological levels of cytokines to support co-transplanted stem cells and resident cells in intervertebral disc therapies.
23.5 Stem Cell Transplantation for Intervertebral Disc Tissue Engineering and Regeneration
The final approach to MSC utilization in intervertebral disc tissue engineering and regeneration involves the construction of a disclike tissue in vitro and its transplantation or the direct delivery of stem cells into the intervertebral disc. Gaetani et al. (2008), Nesti et al. (2008), Driscoll et al. (2011), and See et al. (2011) have all attempted to construct this type of tissue in vitro. To improve the quality of in vitro-reconstructed tissue, Gaetani et al. (2008) constructed a nucleus pulposus-like tissue using a 3D culture of nucleus cells and adipose-derived MSC. To develop a biphasic construct, Nesti et al. (2008) seeded MSC onto a hyaluronic acid–nanofibrous scaffold that consisted of an electrospun, biodegradable nanofibrous scaffold enveloping a hyaluronic acid hydrogel center. The seeded MSCs were induced to undergo chondrogenesis in vitro in the presence of TGFβ for up to 28 days. The cartilaginous hyaluronic acid–nanofibrous scaffold construct resembled a native disc architecturally, with an outer annulus fibrosus-like region and an inner nucleus-like region. Histological and biochemical analyses, immunohistochemistry, and gene expression profiling revealed the time-dependent development of the chondrocytic phenotype of the seeded cells. The cells also maintained the microarchitecture of the native disc. An electrospun nanofibrous scaffold has also been used to examine the native biomechanics of the annulus fibrosus (Driscoll et al. 2011). Previous studies have shown that the tensile and shear properties of the native tissue are dependent on the fiber angle and the sample aspect ratio, respectively, so the effects of changing the fiber angle and the sample aspect ratio on the shear properties of aligned electrospun poly(ε-caprolactone) scaffolds were evaluated. How ECM deposition by the resident MSC modulates the measured shear response was also determined. This team showed that the fiber orientation and sample aspect ratio significantly influenced the response of scaffolds in shear; indeed, the shear properties of both cellular and cell-seeded formulations can match or even exceed the native tissue.
A different approach to tissue engineering the annulus fibrosus was reported by See et al. (2011). They constructed cell sheets from bone marrow MSC and incorporated them onto silk scaffolds to simulate the native lamellae of the annulus fibrosus. They then wrapped the construct around silicone nucleus pulposus, mimicking an intervertebral disc construct, and used a bioreactor to provide compressive mechanical stimulation to the silicone disc. Under static conditions, the MSC sheets remained viable, with no significant change in cell numbers for 4 weeks. A histological analysis showed that the MSC sheets adhered well to the silk scaffolds and glycosaminoglycans were detected within the ECM. The ratio of collagen I to collagen II within the ECM of the MSC sheets also decreased significantly over the period of culture. These results suggest that extensive remodeling of the ECM occurred within the simulated disclike assembly and that this assembly is suitable for the regeneration of the inner annulus fibrosus.
Finally, there have been several attempts to supplement viable cells in the disc by direct stem cell transplantation. The thought behind this concept is the finding that degeneration is initiated by reductions in the numbers, viability, and functions of disc cells, especially those of the nucleus pulposus. Basic in vitro studies have shown that disc cells have a low proliferative capacity and that most cells in the adult human disc are in a senescent state. These facts have led researchers to focus on the idea of transplanting stem cells, which may show transplanted site-dependent differentiation and function to produce a functional ECM.
Sakai et al. (2003) reported the first MSC transplantation study in a rabbit model of disc degeneration. In follow-up studies (Sakai et al. 2005, 2006), they showed that transplanted MSCs survive, proliferate, and differentiate into cells expressing chondroitin sulfate; keratan sulfate; collagen I, collagen II, and collagen IV; HIF1-α and HIF1-β; HIF2-α and HIF2-β; glucose transporter type 1 (GLUT1) and GLUT3; and matrix metalloproteinase 2 (MMP2), proteins that characterize the native nucleus pulposus cells. They also used RT-PCR to quantify the expression of the genes encoding aggrecan, versican, collagen I and II, interleukin 1β (IL1β), IL6, tumor necrosis factor-α, MMP9, and MMP13, thus demonstrating the increased expression of nucleus pulposus cell markers and the downregulated expression of inflammatory genes. Magnetic resonance imaging (MRI) and radiography confirmed the regenerative effects of the procedure, showing that MSC transplanted into degenerating discs in vivo can survive, proliferate, and differentiate into cells that express the phenotype of nucleus pulposus cells, with suppressed inflammatory gene expression. Since then, numerous similar studies have used different animal models and cell carriers and MSC from various sources (Table 23.1) (Crevensten et al. 2004; Zhang et al. 2005; Hiyama et al. 2008; Hoogendoorn et al. 2008; Ganey et al. 2009; Wei et al. 2009a).
Table 23.1
Summary of in vivo animal experimental studies on stem cell transplantation therapy for intervertebral disc degeneration
Stem cell type | Mode | Animal model | Author | Year |
---|---|---|---|---|
Bone marrow MSCs | (Autologous MSCs expanded) | Rabbit (nucleus aspiration) | Sakai et al. | |
Bone marrow MSCs | (MSCs expanded) | Rat (no injury) | Crevensten et al. | |
Bone marrow MSCs | (Allogeneic MSCs expanded) | Rabbit no injury | Zhang et al. | |
Bone marrow MSCs | (Allogeneic MSCs expanded) | Rabbit (nucleus puncture) | Leung et al. | |
Bone marrow MSCs | (Autologous MSCs expanded) | Canine (nucleotomy) | Hiyama et al. | |
Adipose MSCs | (Autologous MSCs expanded) | Goat (ABC chondroitinase) | Hoogendoorn et al. | |
Adipose MSCs | (Autologous MSCs expanded) | Canine (nucleotomy) | Ganey et al. | |
Bone marrow human MSCs | (Xenogeneic MSC expanded) | Rat (no injury) | Wei et al. | |
Bone marrow human MSCs | (Xenogeneic MSC expanded) | Porcine(nucleus aspiration) | Henriksson et al. | |
Synovial MSCs | (Allogeneic MSCs expanded) | Rabbit (nucleus puncture) | Miyamoto et al. | |
Bone marrow MSCs | (Autologous MSCs expanded) | Rabbit (nucleus aspiration) | Yang et al. | |
Bone marrow human MSCs | (Xenogeneic MSC expanded) | Rat (nucleotomy) | Allon et al. | |
Bone marrow MSC + autologous NP cells | (Autologous MSCs expanded) | Rabbit (nucleus aspiration) | Feng et al. | |
Bone marrow MSCs | (Autologous MSCs expanded)
![]() Stay updated, free articles. Join our Telegram channel![]() Full access? Get Clinical Tree![]() ![]() ![]() |