Fig. 19.1
Schema showing a cross section of the intervertebral disc and anatomy of the spinal cord and posterior vertebral processes. (Left) Normal anatomy showing labels identifying the intervertebral disc anulus fibrosus and nucleus pulposus, as well as the nerve roots which come together as spinal nerves as they exit through the intervertebral foramen. (Right) Schema of anatomic changes typical of a posterolateral intervertebral disc herniation, suggesting nerve root impingement (Used under CCL3.0, http://en.wikipedia.org/wiki/File:Cervical_vertebra_english.png)
Intervertebral disc herniation may also occur in association with disc degeneration, wherein degenerated nucleus pulposus fragments migrate into previously established defects in the anulus fibrosus (Moore et al. 1996). A desiccated and fibrous nucleus pulposus is associated with loss of disc height and an increased axial disc bulge with compressive loading (Adams and Roughley 2006); the altered tissue can generate untoward stresses upon the anulus fibrosus leading to tissue fragment extrusion. Thus, while intervertebral disc degeneration is positively associated with disc herniation, it can be difficult to identify the specific contributions of biomechanical, environmental, or genetic factors. Once the tissue is protruded or herniated, there is evidence for increased angiogenesis, macrophage infiltration, and proteinase production in the tissue fragment that can contribute to its resorption over a period of months to years (Komori et al. 1996).
The factors listed above are also believed to play important roles in the etiology and pathophysiology of intervertebral disc herniation (Adams and Roughley 2006; Battie and Videman 2006). Mechanical loading of the lumbar spine in work-related loading conditions may be sufficient to cause disc herniation. Epidemiological studies further suggest a role for mechanical factors and nutrient transport in intervertebral disc herniation with higher incidences of herniation and sciatica associated with obesity, smoking, and heavy physical work (this topic is discussed in considerable detail in Chap. 9) (Battie et al. 2009; Bostman 1993; Heliovaara 1987a, b; Heliovaara et al. 1987a, b). Genetic predisposition to lumbar radiculopathy may also exist, with data suggesting that disc herniation may be associated with genetic mutations in the α2 and α3 chains of collagen IX or the regulatory cytokines interleukin-1β (IL-1β) and interleukin-6 (IL-6) (see Chap. 11).
For intervertebral disc herniation, the first treatment of choice is conservative, unless motor weakness or loss of function is a significant concern. Lifestyle modifications, in particular exercise and physical therapy, can provide symptomatic relief and reduce the need for operative treatment, although they are not generally considered to be disease-modifying therapies (Weinstein et al. 2006a, b). In addition, conventional pharmacological interventions are widely prescribed for sciatica including orally administered anti-inflammatory and opioid analgesics. While more invasive, epidural administration of anesthetics, such as bupivacaine, and/or corticosteroids, like methylprednisolone or triamcinolone, shows some efficacy in providing symptom relief, although again without evidence of disease modification (Buenaventura et al. 2009; Staal et al. 2008).
While nonsurgical care is the first treatment option (see Chap. 15), surgical intervertebral disc excision is the most frequently performed musculoskeletal procedure in the USA, affecting 0.3 % of the population with hospitalization costs of $9.5 billion (Fraser 2009; Ricci et al. 2006). The surgical approach for painful disc herniation is simply to remove the inflammatory material and to unload and decompress the adjacent neural structures (Loupasis et al. 1999). While frequently successful in providing relief, compression-relieving discectomy may not prevent recurrence of pain, prompting the need for additional surgeries, or in 20–60 % of patients, there may be repeat herniations (Weinstein et al. 2006a, b).
19.2 Pathophysiology and Pain of Intervertebral Disc Herniation
Both chemical and mechanical factors are widely believed to contribute to radicular pain subsequent to intervertebral disc herniation (Olmarker 2001). The herniated fragment may impinge upon the exiting spinal nerve and contribute to nerve root compression with many associated deleterious effects. The root consists of both anterior and posterior rootlets exiting from the spinal nerve that combine to form the dorsal and ventral nerve roots containing sensory and efferent fibers, respectively (Fig. 19.2). The roots come together in the region of the neural foramen and continue more distally into the periphery of the spinal nerve to innervate the structures outside of the spinal column. Unlike nerves, the nerve roots are not enclosed by a thick epineural sheath, and so they lack the mechanical resilience of their peripheral counterparts; hence, their structural anatomy places them at particular risk for injurious mechanical loading under intervertebral disc herniation. In addition, the cell bodies of peripheral nerves are housed in the dorsal root ganglion (DRG) and can respond to even slight compression with sustained neuronal activity and pain (Hanai et al. 1996; Hu and Xing 1998; Van Zundert et al. 2006). Intraoperative studies in patients with disc herniation and associated root impingement demonstrate decreases in the amplitude of compound muscle action potentials following electrical stimulation to the affected nerve root (Morishita et al. 2006; Takamori et al. 2011). This work provides direct support for the clinical observation that functional impairment is associated with disc herniation. Reproduction of pain generating straight-leg raises in patients undergoing surgery showed that the amplitude of the evoked action potentials decreased 41 % at as early as 1 min following positioning and decreased by 63 % after 3 min (Takamori et al. 2011). The change in evoked action potential continued to develop through the period of nerve root impingement, demonstrating that time-dependent electrophysiologic responses occur in the nerve root with compression. These changes in neuronal signaling probably contribute to radiculopathy symptoms. Together, the magnitude, duration, and rate of compression of the nerve root modulate both the extent of the local tissue damage and the degree and duration of the pain symptoms (Kobayashi et al. 2005b; Olmarker et al. 1989; Rothman et al. 2010; Rydevik et al. 1991; Winkelstein et al. 2002).
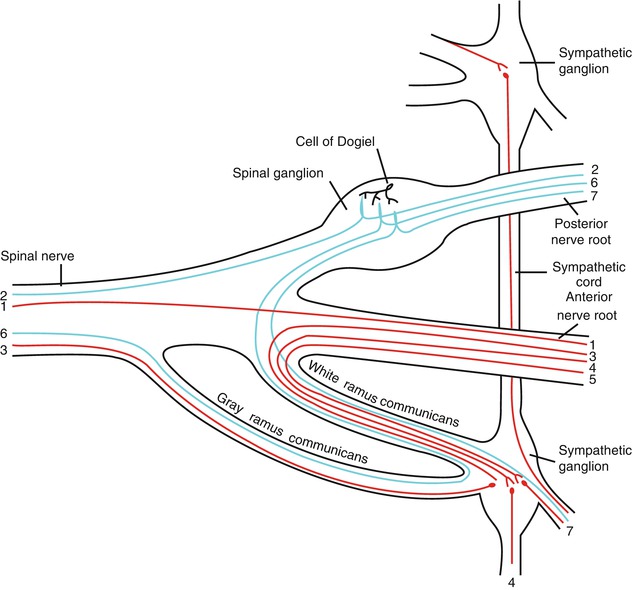
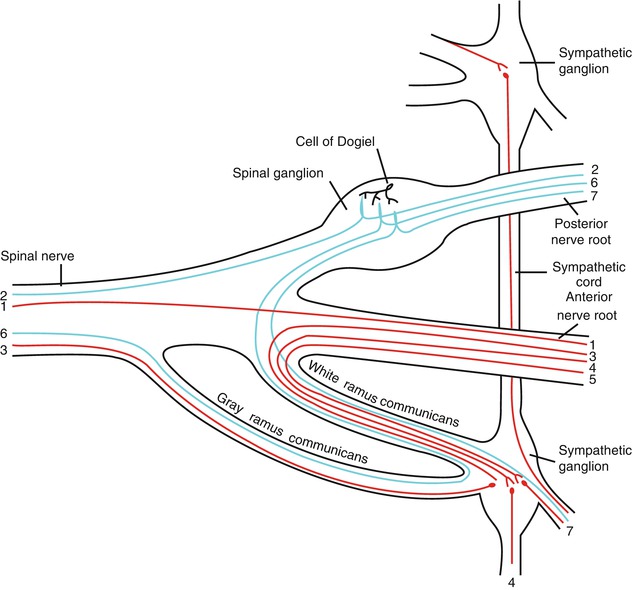
Fig. 19.2
Scheme showing structure of a typical spinal nerve and spinal ganglion. 1 Somatic efferent, 2 somatic afferent, 3, 4, 5 sympathetic efferent, 6, 7 sympathetic afferent (Figure 799 from Gray’s Anatomy (Used under CCL3.0, http://en.wikipedia.org/wiki/File:Gray799.svg))
As indicated above, intervertebral disc herniation can contribute to a type of neuropathic pain that has, as its most common characteristic symptom, radicular pain or radiculopathy. Self-reported pain and disability scales, such as the visual analogue scale (VAS) or Oswestry Disability Index, are often used to provide measures of pain or functional loss associated with disc herniation. Clinically, the straight-leg raise test (or SLR) is considered the most sensitive approach for quantifying the degree of radicular pain originating in the lumbar region, as pain is more pronounced upon elevation of the leg (van der Windt et al. 2010). Similarly, positions that reproduce pain such as forward flexion, hyperextension, and slump are sometimes used to corroborate findings of radicular pain, although imaging is commonly needed to confirm the pain source is related to intervertebral disc herniation. In patients, physical tests of muscle weakness, impaired reflexes, and sensory deficits or hypersensitivity may also be used to detect impairment associated with radiculopathy. These diagnostic and quantitative assessments are relevant to animal models as no diagnostic biomarkers that span human to animal models have yet been developed for intervertebral disc herniation radiculopathy (Brisby et al. 2002; Gajendran et al. 2011; Tokunaga et al. 2010). Tests of hypersensitivity to non-noxious (allodynia) and noxious stimuli (hyperalgesia) serve as surrogate measures of sensory changes with disc herniation and can serve as indicators of neuropathic pain in both human subjects and animals. A heightened response to a light brush of the skin would be considered a sign of mechanical allodynia, while a heightened response to pinch would be considered a sign of mechanical hyperalgesia. Patients presenting with herniation may experience either or both mechanical or/and thermal allodynia, and these serve as important metrics of pain-related behaviors in animal models of intervertebral disc herniation.
Separate from nerve root compression, a herniated disc fragment may evoke an inflammatory and immune response near an affected root (Olmarker and Larsson 1998). Indeed, the herniated tissue fragment is a known generator of many inflammatory mediators and proinflammatory cytokines, such as IL-1α, IL-6, and TNF-α (Table 19.1). Elevated expression of these molecules may activate the immune system and upregulate the expression of proteinases important for fragment resorption; nerve root compression alone may also upregulate the expression of many of these same inflammatory mediators (Kobayashi et al. 2005b). Many mediators are known generators of pain, such that they have become therapeutic targets for new and emerging studies (reviewed at the end of this chapter).
Table 19.1
Molecular mediators of radiculopathy identified in intervertebral disc tissues. Annotated findings in footnotes
Mediator | Notes | Citation |
---|---|---|
TNF-α | 1–3,6 | |
ICAM-1 | 1 | Doita et al. (1996) |
Interleukin-1α | 1,2 | |
Interleukin-1β | 1,3,7 | |
Interleukin-17 | 3 | Shamji et al. (2010) |
Interleukin-4 | 3,5 | |
Interleukin-6 | 1,3,6 | |
Interleukin-8 | 1,2,4 | |
Interleukin-12 | 3,4 | |
Interleukin-20 | 1 | Huang (2008) |
Interferon-γ | 1,3,4 | |
Leukotriene-B4 | ||
Thromboxane-B2 | 1,4,7 | |
Phospholipase A | 1 | Saal (1990) |
Prostaglandin E2 | 1,3 |
19.3 Animal Models of Intervertebral Disc Herniation
To advance therapeutic options that can change disc herniation outcomes, it is of critical importance to understand the molecular mechanisms that regulate pain, muscle changes, and dysfunction. Animal models of herniation have been extensively studied for this purpose. These models fall into two categories: (1) models designed to mimic direct nerve root compression in a well-controlled manner using plungers, constriction, or graded compression (Hou et al. 2003; Kallakuri et al. 2005; Kawakami et al. 2003; Onda et al. 2005; Sekiguchi et al. 2009); (2) models designed to mimic chemical injury that can elicit an inflammatory response through the use of chemical irritants (Colburn et al. 1999; Hashizume et al. 2000a; Hubbard and Winkelstein 2005; Kajander et al. 1996; Kawakami et al. 1994a, b; Maves et al. 1993; Olmarker et al. 1993; Winkelstein and DeLeo 2004); or (3) direct application of nucleus pulposus tissue to the nerve root (Allen et al. 2011; Brisby et al. 2000; Cuellar et al. 2005; Kawakami et al. 1999; McCarron et al. 1987; Olmarker et al. 1997; Olmarker and Myers 1998; Otani et al. 1997; Sekiguchi et al. 2008; Shamji et al. 2009). All of these animal models mimic key features of painful radiculopathy such as limb allodynia or hyperalgesia. The most commonly reported measure of limb hypersensitivity in preclinical models of intervertebral disc herniation is mechanical allodynia, detected using von Frey microfilaments applied to the plantar region of an animal’s paw (Fig. 19.5) (Colburn et al. 1999; Kallakuri et al. 2005; Kobayashi et al. 2005b; Shamji et al. 2009; Rothman et al. 2010; van der Windt et al. 2010). By measuring the frequency or response of withdrawal from a given filament, the sensitivity of a limb to non-noxious mechanical stimuli can be assessed. Mechanical hyperalgesia is also a measure of limb sensitivity and can be determined by recording the time spent grooming following a pinprick or assessing the mechanical pinch force required to initiate an animal response (Randall–Selitto device). These measures have some gross relationships to pain and serve as surrogate measures of underlying neuropathology with intervertebral disc herniation.
Pain or dysesthesia can also elicit changes in grooming, locomotion, or sensorimotor skills in animal models. Pain in disc herniation models has been identified by recording key features of animal behavior over a period of time, such as the frequency of head turns toward a respective limb, leg lifts, duration of spontaneous grooming activity on a given limb, and “wet-dog shakes” (see Chap. 16) (Nakamae et al. 2011; Nilsson et al. 2011; Olmarker 2008; Olmarker et al. 2002, 2003). Alternatively, quantitative measures of gait have been used to identify compensations and disabilities resulting from intervertebral disc herniation (Allen et al. 2011; Shamji et al. 2009), made easier by the recent introduction of digitized gait apparata including the CatWalkTM, TreadscanTM, and DigigaitTM systems (Beare et al. 2009; Berryman et al. 2009; Gensel et al. 2006; Piesla et al. 2009; Vrinten and Hamers 2003). Tracking of spatial and temporal gait parameters, such as the geometric position of the limb and paw ground contact times, can be used to obtain measures such as stride length, step width, toe-out angle, stance times, gait symmetry (a measure of limping), and running velocity (Fig. 19.3). Dynamic data describe forces and moments that occur during a gait cycle and include measures such as ground reaction forces and moments (Crawley 2007; Whishaw and Kolb 2005). These parameters are standard analytical tools in the study of musculoskeletal injury and pathology and are more recently being used to objectively quantify pain-related behaviors and functional losses in animal models of disc herniation. The major models used to study mechanisms that contribute to symptoms of intervertebral disc herniation are reviewed here, followed by an overview of developments in the area of emerging nonsurgical therapies.
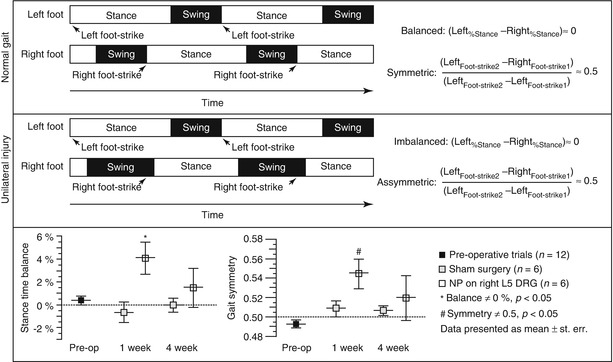
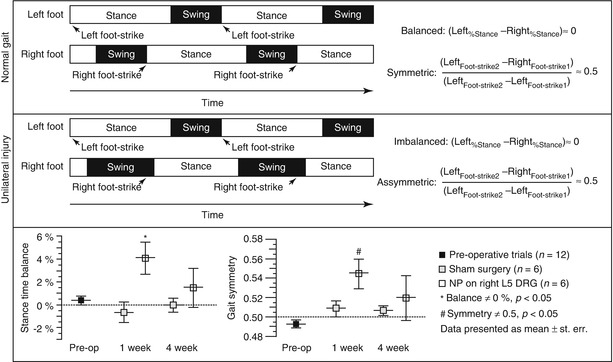
Fig. 19.3
Definitions of temporal parameters obtained from quantitative gait analysis. Rats freely ambulate across a clear gait chamber while digital video is acquired for determination of geometric positions of both hindpaws during each gait cycle. As shown, parameters of stance time, gait symmetry, as well as self-selected velocity, stance width, stride length, and more can be measured. (Top) Schema of a normal gait sequence wherein stance times are similar on the left and right hind limbs and a right foot-strike event occurs at the midpoint between two left foot-strike events. (Middle) For unilateral right limb injury, increased time is spent on the left limb and decreased time is spent on the right limb, as seen relative shifts in stance times. Also, the foot-strike sequence becomes syncopated in time, with the right limb foot strike occurring past the midway point of two left limb foot strikes. (Bottom) Data for stance time imbalance and gait symmetry of rats in a nucleus pulposus injury model of radiculopathy as compared to sham controls (Plotted from Hwang et al. (2012), used under CCL3.0)
19.3.1 Mechanical Factors in Intervertebral Disc Herniation
Nerve root compression in association with disc herniation can result from acute insult to the axonal, connective, and vascular tissues of the nerve root and initiate a cascade of related and integrated neuronal, inflammatory, and degenerative changes (Kobayashi et al. 2004b; Rydevik et al. 1984, 1994; Winkelstein et al. 2002). From this perspective, mechanical injury and inflammatory injury are not unique, nor unlinked, events. Nerve root compression following acute mechanical loading can induce long-term nerve root pathophysiology, such as edema, inflammation, and thickening of connective tissues (Beck et al. 2010; Jancalek and Dubovy 2007; Kobayashi et al. 2004b; Mosconi and Kruger 1996), as well as the development of evoked pain via modified communication with the spinal cord. Following mechanical trauma and compression, injured axons exhibit axonal swelling, loss of cytoskeleton proteins, separation and disorganization of the myelin sheath, loss of axonal transport, Wallerian degeneration, and a decrease in axon packing density (Guertin et al. 2005; Jancalek and Dubovy 2007; Kobayashi et al. 2004a, b, 2005a, b, c, d; Mosconi and Kruger 1996; Myers et al. 1993). Like functional changes in neurons during and after compression, degenerative changes in axons develop at later times and are also dependent on the magnitude of the compression (Hubbard et al. 2008b; Kobayashi et al. 2005b; Nicholson et al. 2011).
Cells that respond to injury include microglia (resident macrophages in the central nervous system) and astrocytes. These cells have many roles in both the peripheral and central nervous systems, including the maintenance of homeostasis at neuronal synapses. Both types of glial cells respond to injury by changing their morphology, proliferating, upregulating cell surface markers, and releasing several inflammatory mediators (Cao and Zhang 2008; DeLeo et al. 2004; Saab et al. 2008; Suter et al. 2007). A peripheral stimulus by some neurotransmitters/neuromodulators (e.g., excitatory amino acids, substance P, ATP) (Cao and Zhang 2008; Marriott 2004) can activate early release of proinflammatory cytokines as well as nitric oxide, prostaglandins, and nerve growth factor (DeLeo et al. 2004; Inoue 2006). These mediators, in turn, induce an exaggerated release of neurotransmitters from presynaptic neurons, sensitize the postsynaptic membrane, activate neighboring astrocytes, and enhance microglial activity (DeLeo et al. 2004; Inoue 2006). This positive feedback sustains the release of pain mediators, facilitating the development of neuronal hypersensitivity and can lead to the persistent pain that is often associated with a herniated disc or inflamed nerve root. Following such neural insults, spinal glial cells become activated and modulate other immunologic changes via cytokine and growth factor production, leading to persistent pain (DeLeo and Yezierski 2001; Hashizume et al. 2000a; Obata et al. 2004; Winkelstein et al. 2001a). In particular, greater nerve root compression leads to increased activation of spinal astrocytes that is apparent as early as 1 day and is sustained in parallel with persistent symptoms of mechanical allodynia (Rothman and Winkelstein 2007). These observations suggest that spinal astrocytes may directly respond to the changes in the dorsal horn that are induced by damaged primary afferents (Hogan 2007; Sapunar et al. 2005) (Fig. 19.4).
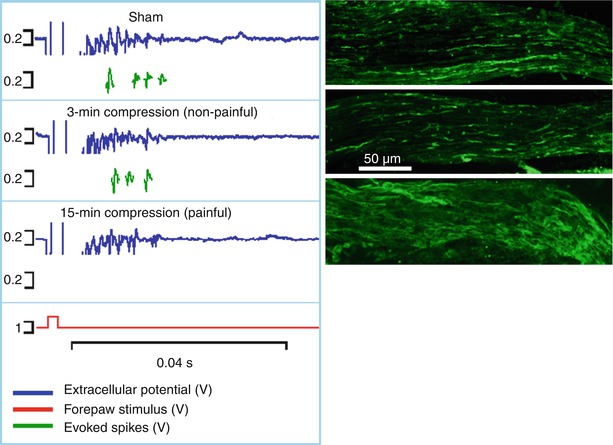
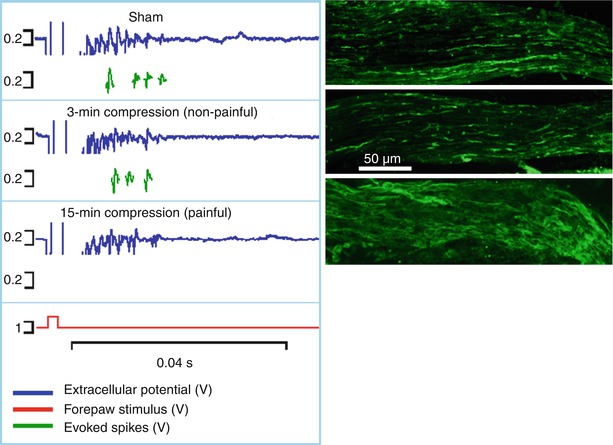
Fig. 19.4
Both the spinal extracellular potential (blue trace) and number of spikes evoked by electrical stimulus (green traces) generated following a nerve root compression are modified by the duration of the applied compression. The longer duration (15 min) compression that produces pain symptoms also causes an abolishment of evoked responses and decrease in EC response. In addition, that loading scenario also produces axonal swelling in the unmyelinated nerve fibers of the root that is absent in the 3-min (non-painful) compression
Severe axonal injury can also induce Wallerian degeneration of the axonal process distal to the cell body (Stoll and Jander 1999; Stoll and Muller 1999). For the central axons of primary afferents, which make up the dorsal nerve root, Wallerian degeneration can occur proximal to the site of injury (Hubbard and Winkelstein 2008; Kobayashi et al. 2008). Axonal degeneration, marked by neurofilament degradation and loss of axonal integrity, is evident as early as 15 min after trauma, but is more commonly present at time points in the order of weeks (Kobayashi et al. 2008; Ramer et al. 2004). The extent of degeneration is modulated by the mechanics of the insult and is associated with persistent pain and hypersensitivity after a compression to the nerve root (Dyck et al. 1990; Hubbard et al. 2008a, b; Kobayashi et al. 2008; Nicholson et al. 2011) (Fig. 19.5). Disruption to the axonal structure has been found to be more pronounced for greater loads applied for longer durations (Dyck et al. 1990; Hubbard et al. 2008a; Kobayashi et al. 2005a, 2008; Nicholson et al. 2011). Further, the extent of damage and Wallerian degeneration of the axons in the compressed nerve root is directly related to the development of persistent hypersensitivity (Hubbard et al. 2008a, b; Hubbard and Winkelstein 2008).
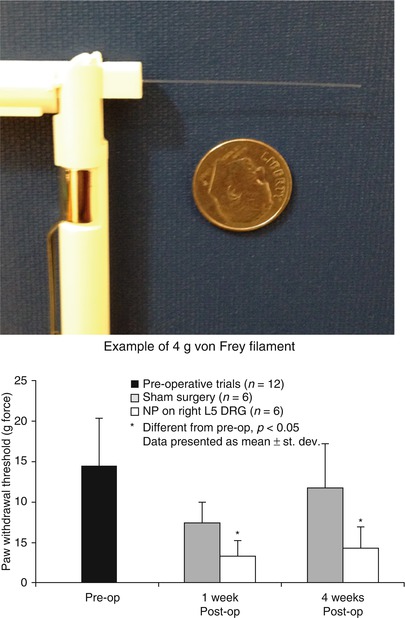
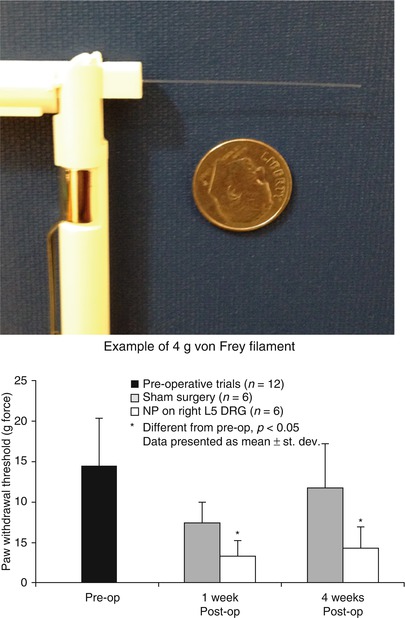
Fig. 19.5
Apparata and typical results for measuring mechanical allodynia in a rodent model of radiculopathy. (Left) von Frey filaments are logarithmically graded (e.g., 4g as shown) by buckling load upon compressive application. (Right) When filaments of varying strengths are applied to the hindpaw of a rat following placement of nucleus pulposus tissue upon the lumbar dorsal root ganglion (DRG), paw withdrawal is recorded over multiple trials and filaments. Data for paw withdrawal threshold of rats in an NP injury model of radiculopathy as compared to sham controls. Data show that nucleus pulposus placement upon a naïve DRG induces a paw withdrawal at lower filament strength, indicative of a persistent sensitivity to non-noxious stimuli (mechanical allodynia) (Plotted from Hwang et al. (2012), used under CCL3.0)
The severity of nerve root injury and the intensity of pain after nerve root injury inflammation strongly relate to neuropeptide depletion in the DRG and spinal cord together with axonal degeneration (Hubbard et al. 2008a, b; Rothman et al. 2005). For example, persistent allodynia, due to higher magnitudes of dorsal nerve root compression, is associated with greater sensitivity at 1 week or later (Hubbard et al. 2008a, b). This is accompanied by a corresponding depletion of the nociceptive neuropeptide, substance P, in the DRG that similarly varies with the magnitude of the initiating compressive load (Hubbard et al. 2008b; Kobayashi et al. 2005b). Spinal expression of another potent neuropeptide for regulating pain, calcitonin gene-related peptide (CGRP), also decreases with increased painful loading to the nerve root (Hubbard et al. 2008a, b). Together with the effects of neural trauma described above, these mechanically deleterious events can dramatically contribute to reduced transport of neuropeptides and neurotrophic factors from neurons, where these factors are synthesized, to their release from the presynaptic terminals in the spinal cord.
In animal models, the magnitude, duration, and rate of the nerve root compression have been shown to modulate the extent of the local tissue damage to the root and the degree and duration of the pain-related behaviors (Kobayashi et al. 2005a; Olmarker et al. 1989; Rothman et al. 2010; Rydevik et al. 1991; Winkelstein et al. 2002). In rodent models of nerve root compression, elevated magnitudes of compression increased the levels of mechanical allodynia and reduced axonal transport in the compressed root (Kobayashi et al. 2005a; Winkelstein et al. 2002). Although animal models of nerve root compression have shown sustained mechanical hypersensitivity in the affected limb (Colburn et al. 1999; Hashizume et al. 2000a; Kobayashi et al. 2005a; Winkelstein and DeLeo 2004; Winkelstein et al. 2002), mechanical hypersensitivity can also be produced when the nerve root is compressed for times as short as 2 s (Sekiguchi et al. 2003, 2009). Moreover, lumbar nerve root compression produces an immediate change in evoked signal conduction along the fibers of the compressed root (Fumihiko et al. 1996; Morishita et al. 2006; Pedowitz et al. 1992; Rydevik et al. 1991; Takahashi et al. 2003). Both compression rate and magnitude contribute to edema production in the nerve root, such that the magnitude of pressure required to produce edema decreases for higher loading rates (Hubbard et al. 2008b; Hubbard and Winkelstein 2008; Nicholson et al. 2011, 2012; Olmarker et al. 1989; Rothman et al. 2010; Rydevik et al. 1991). Moreover, specific loading parameters, such as magnitude and duration, likely play a role in modulating electrophysiologic responses. Animal models of nerve root compression in the cauda equina demonstrate that evoked neuronal signaling is altered during and after compression (Fumihiko et al. 1996; Garfin et al. 1990; Pedowitz et al. 1992; Rydevik et al. 1991) and decreases in the amplitude of electrically evoked compound nerve action potentials due to cauda equina compression may persist after removal of the compressive force (Pedowitz et al. 1992; Rydevik et al. 1991). These changes are corroborated by measurements in human subjects with intervertebral disc herniation that similarly show changes in evoked nerve action potentials upon straight-leg raise.
The results of studies of mechanically compressed nerve roots together with the widespread molecular changes can be integrated into a generalized schema. As early as 1 h following even a transient nerve root compression that is sufficient to produce persistent behavioral sensitivity, inflammatory cytokines, IL-6 and TNF-α, and mRNA expression levels are elevated in the ipsilateral DRG and also in the spinal cord (Rothman et al. 2009b). Within 1 day of that event, behavioral sensitivity develops along with hallmarks of spinal inflammation, including activation and proliferation of microglia (Rothman et al. 2009a). By 7 days after injury, axons of the injured nerve root show signs of degeneration, and the spinal inflammation becomes even more pronounced, and both astrocytes and microglia become activated (Hubbard and Winkelstein 2005, 2008). These changes together with the decrease in neuropeptides in the spinal cord at this same time point (Hubbard et al. 2008b) may lead to alterations in neuronal signaling in the spinal cord following a painful injury.
19.3.2 Role of Chemical and Inflammatory Mediators in Disc Herniation
As mentioned above, contributing to a cascade of chemical injuries at the affected nerve are elevated levels of inflammatory mediators, infiltration of macrophages, and activation of glial cells. To further examine these effects, animal models have been developed that mimic features of both inflammation and immune system function in intervertebral disc herniation. Both behavioral hypersensitivity and widespread immune responses are produced when chromic gut suture pieces are placed in contact with the nerve root without any mechanical perturbation (Colburn et al. 1999; Hashizume et al. 2000a; Hou et al. 2003; Hubbard and Winkelstein 2005; Kajander et al. 1996; Kawakami et al. 1994a, b; Maves et al. 1993; Murata et al. 2004a, b; Olmarker et al. 1993; Rothman and Winkelstein 2007; Rutkowski et al. 2002; Winkelstein and DeLeo 2004). Gut suture ligation simultaneously compresses the nerve root by ligation, while the chromic salts and pyrogallol in the suture serve as irritants (Colburn et al. 1999; Hashizume et al. 2000a; Kajander et al. 1996; Kawakami et al. 1994a, b; Maves et al. 1993; Robinson and Meert 2005; Winkelstein and DeLeo 2004; Xu et al. 1996). A consistent response to the chromic gut suture by the nerve root damage is the induction of thermal hyperalgesia (decreased latency to withdraw from thermal stimuli) that is transient and dose dependent; this form of hyperalgesia is not consistently present in intervertebral disc herniation models that mimic the compressive stimuli alone (Maves et al. 1993; Yamamoto and Nozaki-Taguchi 1995). The mechanisms governing the neuronal responses to chromic gut salts appear to be related to early immune activation characterized by Schwann cell proliferation, macrophage infiltration, and microglial activation. Along with these changes are molecular events including a depleted neuropeptide expression and an early expression of cell adhesion molecules such as ICAM-1 and PECAM. These adhesion molecules would serve to promote recruitment of circulating monocytes to the injured nerve root (Chang and Winkelstein 2011; Hashizume et al. 2000a; Rothman et al. 2010; Rutkowski et al. 2002; Xu et al. 1996; Yamamoto and Nozaki-Taguchi 1995). These studies indicate that, despite similarities in sensitivity to chromic gut suture and mechanical compression, differences in the pattern of hypersensitivity and molecular changes may reflect only limited injury etiologies. Nonetheless, use of chromic gut sutures as a chemical injury model for nerve root damage is popular for evaluating treatments that can interfere in the perception of disc herniation-related pain.
Soft tissues of the disc are believed to be immune privileged, in that the internal structures of the intervertebral disc do not come into contact with the systemic circulation under normal conditions and express the Fas ligand that can promote an autoimmune response. With degeneration and damage, the internal structures of the intervertebral disc can come in contact with adjacent tissues, producing an immune response that is the subject of extensive investigation. Intervertebral disc tissues contain high levels of CD68+ immunoreactive macrophages, as well as lesser quantities of T and B lymphocytes (Doita et al. 1996; Freemont et al. 2002; Gronblad et al. 1994; Roberts et al. 2006; Shamji et al. 2010). In addition, the disc tissues secrete numerous proinflammatory cytokines and inflammatory mediators, such as interleukin-1α (IL-1α), interleukin-6 (IL-6), interleukin-17 (IL-17), and tumor necrosis factor-α (TNF-α); these cytokines may be produced by primary disc cells or by infiltrating monocytes (Bachmeier et al. 2009; Burke et al. 2002; Le Maitre et al. 2007; Murata et al. 2004a; Saal 1995; Shamji et al. 2010; Weiler et al. 2005, 2011) (Table 19.1). Numerous studies have documented the molecular effects of the herniated discal tissues (Mulleman et al. 2006a, b). Models used for these studies have included harvest of tail nucleus pulposus tissue for placement at a lumbar nerve root or cauda equina (Allen et al. 2011; Aoki et al. 2002; Brisby et al. 2000; Cuellar et al. 2005; Kawakami et al. 1999; Shamji et al. 2009; Skouen et al. 1999; Yabuki et al. 1998) or lumbar disc puncture to promote nucleus pulposus herniation (Olmarker et al. 1998; Olmarker and Myers 1998; Otani et al. 1997). Physiological changes noted in these models include reduced nerve conduction velocities, lowered endoneurial pressure for dorsal horn or sensory neurons, and elevated nitric oxide synthase activity in spinal nerve roots at 1–4 weeks following exposure to discal tissues. In addition, there is increased expression of neurotrophins, IL-1β, TNF-α, phospholipase-1, and/or nitric oxide synthase in the applied nucleus pulposus tissues or in cell bodies and intercellular domains around the DRG (Kallakuri et al. 2005; Kawakami et al. 1999; Murata et al. 2004a; Onda et al. 2002). Disc-associated cytokines (IL-1β, TNF-α, IFN-γ) applied directly to nerve roots, as opposed to cytokines secreted by nucleus fragments, have also been shown to induce electrophysiological changes, consistent with a heightened sensitivity (Ozaktay et al. 2002; 2006). It is likely that these cytokines bind to receptors for TNF-α and IL-1β on the sensory neurons (Binshtok et al. 2008; Verri et al. 2006). While these findings clearly indicate a role for inflammation and inflammatory mediators in regulating neuronal sensitivity in intervertebral disc herniation, it must be noted that many changes in the nucleus pulposus-induced or chemical injury models of radiculopathy overlap with those reported for direct compression neuropathy.
As with direct nerve root compression or chromic gut suture exposure, application of nucleus pulposus tissue to a naïve nerve root induces limb hypersensitivity that is largely characterized by a mechanical allodynia (Hou et al. 2003; Mulleman et al. 2006a, b) (Fig. 19.5). Many studies of nucleus pulposus-induced radiculopathy following disc puncture to induce nucleus herniation or placement of nucleus pulposus tissue upon the nerve root report evidence of allodynia as early as 2 days that may persist out to 3 weeks (Kawakami et al. 1999; Obata et al. 2002). Early studies also report functional changes following nucleus pulposus-induced radiculopathy, including altered footprints and visual evidence of limping, paw lift, or rotation of the head (Olmarker et al. 1998, 2002). With the use of quantitative gait analysis, our laboratories have further demonstrated gait asymmetries between ipsi- and contralateral hind limbs out to 3 or 4 weeks postoperatively, indicative of limping (Shamji et al. 2009) (Fig 19.3). Static and dynamic gait analyses indicate that animals with radiculopathy bear less weight on their affected hindpaw in both stance and during locomotion (Allen et al. 2012).
The findings discussed above suggest that radiculopathy induced by DRG exposure to nucleus pulposus tissue, as a model of intervertebral disc herniation, can repeatedly mimic key characteristics of prolonged pain sensitivity in the human patient (Allen et al. 2011; Shamji et al. 2009). The finding of persistence of sensitivity supports the notion that the molecular responses of an inflamed disc may influence sensory changes during intervertebral disc herniation. The persistent sensitivity changes, extending beyond fragment removal or resorption, imply that the widespread responses imitated in the CNS and even systemically are not ameliorated. Once initiated, the neuroimmune cascade involves activation of many nonneuronal cells in the peripheral tissues (DeLeo and Yezierski 2001; Julius and Basbaum 2001; Moalem and Tracey 2006). These resident cells, including mast and Schwann cells, release mediators such as histamine, prostaglandins, cytokines, and chemokines that also lead to the recruitment of other infiltrating immune cells (e.g., neutrophils, macrophages, lymphocytes) (DeLeo and Yezierski 2001; Moalem and Tracey 2006; Verri et al. 2006). Proinflammatory cytokines also trigger the release of many other inflammatory mediators that can sensitize nociceptors, further maintaining neuronal excitability and sensitization and leading to dysfunction and pain (Moalem and Tracey 2006; Verri et al. 2006). Nonetheless, the persistence of pain observed in some human subjects following intervertebral disc herniation that may continue for months and even years has not been replicated in these animal models, where mechanical allodynia or thermal hyperalgesia can recover to control or preoperative values at later times after surgery. Additional studies of CNS sensitization in human and animal models of disc herniation are needed to better understand the role of these important pathway changes in the pathogenesis of disc pain.
Taken together, with knowledge gained from direct nerve root compression studies, it becomes clear that many of the sensitization and functional changes associated with disc herniation may reflect contributions from both compressive trauma as well as inflammatory agents in the disc. While somewhat intuitive, the more severe nerve root injuries (with greater degrees of tissue impingement) produce more pain-associated behavioral sensitivity than those injuries with less tissue compression (Hubbard et al. 2008b; Hubbard and Winkelstein 2005; Winkelstein et al. 2001b, 2002). This graded relationship has been shown to hold true regardless of the absence or presence of molecular challenges (Winkelstein and DeLeo 2004). Indeed, evidence that the duration of the mechanical trauma modulates several different pain pathways strongly supports the concept that a more permanent mechanical insult would produce a similar or even more robust deleterious clinical effect. Based on these understandings, clinical interventions, and specifically nonsurgical therapies, are focused on alleviating the persistent sensitivity that is associated with a transient or more chronic compressive trauma to the nerve root and the associated inflammatory events. This topic is briefly covered in the next section on emerging pharmacological approaches to treat intervertebral disc herniation-associated radiculopathy.
19.4 Emerging Therapies for Intervertebral Disc-Associated Radiculopathy
As mentioned in Sect. 19.1, in the absence of motor weakness or related loss of function, conservative care is the preferred treatment for a patient presenting with pain and symptoms of radiculopathy secondary to an intervertebral disc herniation. Conservative care most frequently involves lifestyle modifications as well as orally administered nonsteroidals or opioid analgesics. Commonly prescribed selective or nonselective nonsteroidal drugs include ibuprofen, indomethacin, diclofenac, piroxicam, diflunisal, and celecoxib, few of which have demonstrated significant effects on radicular pain associated with disc herniation (Chou and Huffman 2007) (also see Chap. 15). In animal models, indomethacin, ibuprofen, diclofenac, and celecoxib have been shown to reverse allodynia following peripheral or nerve root compression and may work by lowering prostaglandins and inhibiting the resulting decreases in nerve conduction velocity and decreased intraneural blood flow (see Table 19.2). Epidural administration of anesthetics such as bupivacaine and/or corticosteroids, including methylprednisolone, is also widely used for treatment of symptoms with radiculopathy, and they appear to have some efficacy as measured with both objective (e.g., straight-leg raising test) and self-reported outcomes (e.g., VAS) (Buenaventura et al. 2009; Staal et al. 2008). Methylprednisolone and other corticosteroids may work by inhibiting the increase in endoneurial vascular permeability and the decrease in nerve conduction velocity that follows injury to the nerve root upon exposure to nucleus pulposus tissue or chemical injury (Byrod et al. 2000; Olmarker et al. 1994). Regardless of the mechanism, use of these NSAIDs and corticosteroids is generally considered safe and a first line of treatment for patients presenting with pain from intervertebral disc herniation.
Table 19.2
Summary of compounds under investigation for treatment of IVD herniation-associated radiculopathy
Agent | Route | Model description | Reference | Observations |
---|---|---|---|---|
Anti–inflammatories | ||||
Indomethacin | Oral | Canine lumbar nerve injury | Arai et al. (2004) | Reversed changes in intraneural blood flow and nerve conduction |
Ketoprofen | Systemic (i.m.) | Porcine nerve root constriction or NP tissue placement | Cornefjord et al. (2001) | Partly reversed decreased nerve conduction velocity in constriction but not NP exposure |
Diclofenac | Systemic (i.m.) | Porcine nerve root constriction or NP tissue placement | Cornefjord et al. (2001) | Partly reversed decreased nerve conduction velocity |
Ibuprofen | Oral | Rat sciatic nerve compression injury | Schafers et al. (2004) | Reduced mechanical allodynia at short times after injury, reduced PGE2 levels in nerve and DRG |
COX-2 inhibitor celecoxib | Oral | Rat sciatic nerve compression injury | Schafers et al. (2004) | Reduced mechanical allodynia at short times after injury, reduced PGE2 levels in nerve |
NO synthase inhibitor (L-NAME) | Intrathecal | Rat lumbar DRG compression injury | Ding et al. (2010) | Some reversal of thermal hyperalgesia, decreased nitrite in DRG |
Prostaglandin E2 receptor antagonist (EP1-RA) | Oral | Rat exposure of lumbar DRG to NP | Sekiguchi et al. (2011) | Reduced mechanical allodynia, attenuated increased activating transcription factor-3 (ATF3) immunoreactive positive cells induced by NP |
Thromboxane A2 synthetase inhibitor | Epidural | Rat exposure of lumbar DRG to NP | Kawakami et al. (2001) | Reduced mechanical allodynia |
Leukotriene B4 receptor antagonist (LTB4 receptor antagonist) | Epidural | Rat exposure of lumbar DRG to NP | Kawakami et al. (2001) | Reduced mechanical allodynia |
COX-2 antibody | Intrathecal | Rat exposure of lumbar DRG to NP | Ohtori et al. (2004) | Reduced mechanical allodynia |
Neuronal receptor modifiers | ||||
NMDA receptor antagonist, MK-801 | Intraspinal or systemic (i.p.) | Rat spinal nerve or sciatic nerve constriction injury | Reversed molecular changes in CNS, partly reversed motor changes | |
Gabapentin | Systemic i.p. or local (perineural) delivery | Rat sciatic nerve or lumbar nerve root constriction injury | Some reversal of mechanical allodynia
![]() Stay updated, free articles. Join our Telegram channel![]() Full access? Get Clinical Tree![]() ![]() ![]() |