Fig. 20.1
Representative light microscopic images of the aging sand rat lumbar disc: (a) Lumbar disc at 3 weeks of age: image shows nucleus pulposus filled with notochordal cells, annulus, and end plates (scale = 200 μm). (b) Higher-resolution view of the syncytial network of the notochord (scale = 20 μm). (c) The nucleus pulposus in a 3-month-old animal. Note that the notochordal cells have begun to fragment (scale = 50 μm). (d) Annulus-nucleus transition zone lacks clear definition in this specimen from an 11.7-month-old animal (scale = 50 μm). (e, f) Images of the nucleus from an 11.7-month-old (e) and 17-month-old (f) animals show progressive degeneration of matrix and cells (scale on E = 50 μm and on F = 200 μm). (g–j) Low magnification images of lumbar discs showing herniations in the dorsal portions of lumbar discs. Note the greatly narrowed nucleus regions. (g) 18-month-old animal (scale = 200 μm). (h) 28-month-old animal with regions of sequestered nucleus pulposus matrix isolated in the herniation region (scale = 200 μm). (i) 30-month-old animal. (scale = 500 μm). (j) 36-month-old animal (scale = 500 μm) (SP spinal cord, Masson-trichrome staining)
Analyses of live and dead cells in the aging sand rat have also been carried out using fluorescent markers (Gruber et al. 2008a). This work utilized animals aged 2–6, 13–19, and 26–38 months of age. The percentages of dead cells for the entire annulus were 46.1, 48.1, and 76.8 %, respectively, for the three age groups studied. The percentage of dead cells correlated significantly with end plate bone mineral density (p < 0.02). (Bone mineral density studies are discussed in greater detail below.) This study also confirmed the progressive death of notochordal cells in the nucleus during aging as previously noted in morphologic studies.
Sand rat disc tissue has also been extremely valuable when used in parallel with human disc tissue for specialized studies and for immunohistochemical investigations. Like human disc tissue (Gruber and Hanley 1998), sand rat discs show the presence of programmed cell death (apoptosis) and cell senescence (Gruber et al. 2007b). Immunolocalization studies in the sand rat disc have shown patterns similar to those present in human discs for the presence of thrombospondin (Gruber et al. 2006b), myocilin (Gruber et al. 2006a), and pregnancy-associated plasma protein-A (PAPP-A) which unmasks insulin-like growth factor binding protein-4 in the extracellular matrix (Gruber et al. 2008b). The sand rat disc shows a pattern of localization of brain-derived neurotrophic factor (BDNF) similar to that seen in the human disc (Gruber et al. 2008c). Asporin, a member of the family of small leucine-rich proteoglycan (SLRP) family which has been reported to have a genetic association with osteoarthritis, is present in both the human and sand rat disc (Gruber et al. 2009b). Periostin, a matricellular protein in the fasciclin family expressed in tissues subjected to constant mechanical stress, has also been shown to be present within both the sand rat and human disc (Gruber et al. 2011a).
20.3.2 Radiology and Micro-CT
20.3.2.1 Lumbar Spine
The sand rat spine contains seven lumbar discs (Table 20.1). Radiologic characterizations of degenerating discs in both cross-sectional and prospective groups of animals have been performed (Gruber et al. 2002a, 2007a) (Table 20.1). Cross-sectional studies showed significant age-related changes comprising irregular disc margins, disc wedging, disc narrowing, end plate and ligament calcification, and osteophyte formation. Figure 20.2 illustrates radiologic changes at 12, 29, and 30 months of age, including wedging (Fig. 20.2a, arrow), osteophyte formation (Fig. 20.2b), and bone bridges between osteophytes (Fig, 20.2c). Males were more likely to develop osteophytes than were females. There was no gender difference for other radiographic features.
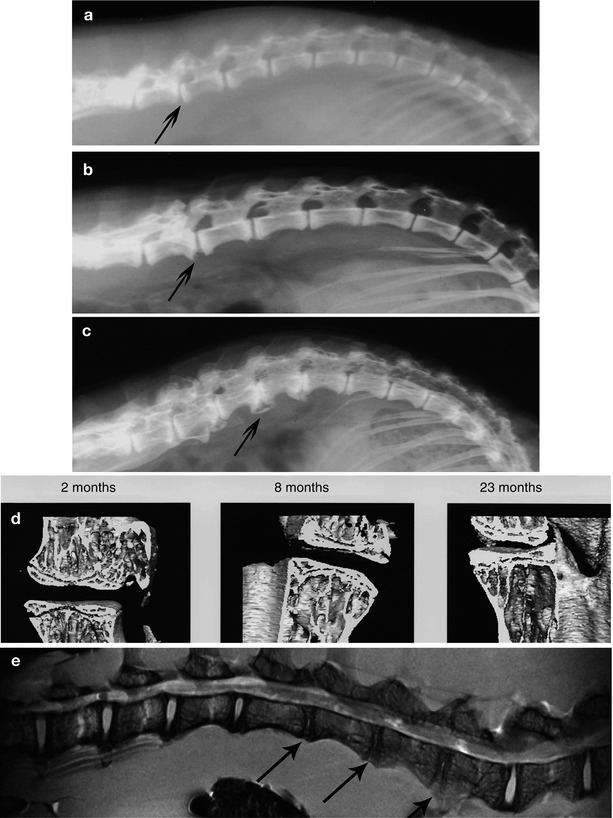
Table 20.1
Analysis of the incidence of radiologic abnormalities (%) by age group
Group 1: 1–3.9 months (n = 36) | Group 2: 4.0–11.9 months (n = 18) | Group 3: 12–23.9 months (n = 19) | Group 4: 24–46 months (n = 26) | P valuea | |
---|---|---|---|---|---|
Narrowing | |||||
L1–2 | 4.0 | 5.8 | 38.8 | 34.6 | 0.01 |
L2–3 | 29.4 | 17.6 | 55.5 | 42.3 | NS (0.08) |
L3–4 | 29.4 | 35.2 | 55.5 | 88.4 | <0.001 |
L4–5 | 35.2 | 47.0 | 61.1 | 88.4 | 0.0005 |
L5–6 | 38.2 | 41.1 | 66.6 | 96.1 | <0.001 |
L6–7 | 50.0 | 94.1 | 88.8 | 100.0 | <0.001 |
L7–S | 44.1 | 82.3 | 88.8 | 100.0 | <0.001 |
Wedging | |||||
L1–2 | 11.7 | 5.8 | 33.3 | 26.9 | NS (0.08) |
L2–3 | 14.7 | 29.4 | 55.5 | 46.1 | 0.01 |
L3–4 | 29.4 | 41.1 | 50.0 | 65.3 | 0.04 |
L4–5 | 55.8 | 70.5 | 72.2 | 88.4 | NS (0.056) |
L5–6 | 70.5 | 70.5 | 94.4 | 100.0 | 0.005 |
L6–7 | 64.7 | 76.4 | 83.3 | 92.3 | NS (0.075) |
L7–S | 82.3 | 70.5 | 88.8 | 100.0 | 0.04 |
End plate calcification | |||||
L1–2 | 2.9 | 100.0 | 44.4 | 100.0 | <0.001 |
L2–3 | 2.9 | 100.0 | 61.1 | 96.1 | <0.001 |
L3–4 | 2.9 | 29.4 | 83.3 | 96.1 | <0.001 |
L4–5 | 8.8 | 58.8 | 88.8 | 100.0 | <0.001 |
L5–6 | 26.4 | 70.5 | 100.0 | 100.0 | <0.001 |
L6–7 | 47.0 | 94.1 | 100.0 | 100.0 | <0.001 |
L7–S | 47.0 | 82.3 | 100.0 | 100.0 | <0.001 |
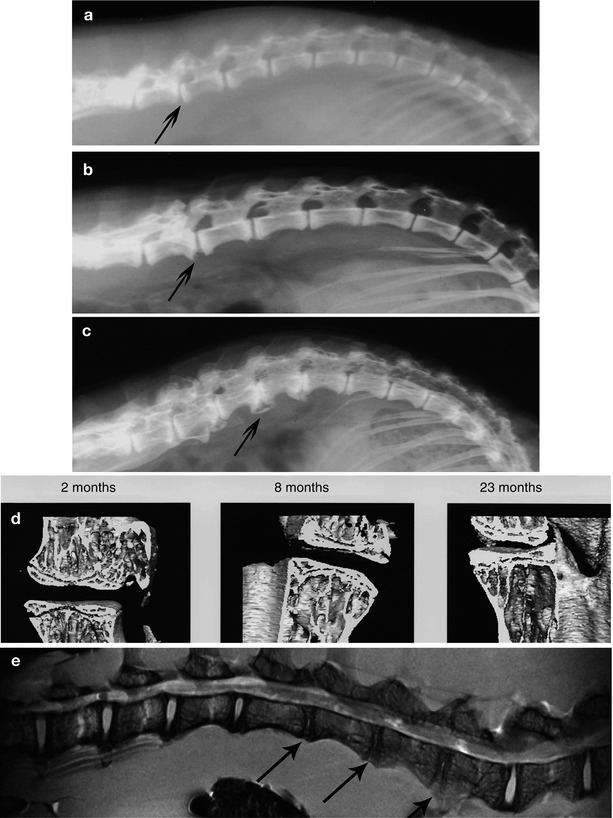
Fig. 20.2
(a–c) Representative radiographic images of the lumbar spine showing wedging (arrow, a), osteophyte formation (arrow, b), and bony bridging across osteophytes (arrow, c). (a) 12-month-old animal; (b) 29-month-old animal; (c) 30-month-old animal. (d) Micro-CT models with cuts revealing interior features of the end plate in 2-, 8-, and 23-month-old animals. Note the progressive loss of porosity in end plates. (e) High-resolution spin-lock (T1r) image of the lumbar spine representative of degenerative changes in older animals. Arrows mark disc space narrowing and loss of proteoglycan content (Image courtesy of Dr. Ravinder Regatte, Depts. of Orthopaedic Surgery and Radiology, New York University School of Medicine)
In the prospective study, 22 sand rats were followed with monthly X-rays from age 2 to 12 months; 11 were males and 11 were females (Gruber et al. 2002a). Statistical analysis showed that wedging, narrowing, end plate calcification, and irregular disc margins were all significantly more common at 12 months of age than at 2 months of age (p = 0.0001). Tests for differences in radiologic disc features related to gender were performed at 2, 3, 6, and 12 months. Males showed a statistically greater incidence (%) of wedging at 6 months (6.4 ± 1.6 vs. 4.8 ± 1.1, p = 0.024) and at 12 months (7.8 ± 0.6 vs. 6.3 ± 1.3, p = 0.004) than females. Narrowing was more frequent in males at age 2 months (1.5 ± 1.6 vs. 0.3 ± 0.5, p = 0.028) and age 6 months (5.8 ± 1.8 vs. 4.1 ± 1.6, p = 0.025) than in females. End plate calcification was more common in 2-month-old males than females (1.8 ± 1.5 vs. 1.2 ± 1.7, p = 0.028). Females, however, showed a greater incidence of end plate calcification at 6 months of age than did males (5.5 ± 1.2 vs. 3.9 ± 0.2, p = 0.009). No gender differences were found for irregular disc margin incidence at 2, 3, 6, or 12 months of age.
Lumbar spine radiographs showing degenerative features in the sand rat have been utilized for development of an automated computer-assisted procedure that analyzed digitized X-rays (Wilson et al. 2003). Techniques were developed to automate the quantitative processing of vertebral radiographic images that may be applicable to modeling of human disc degeneration. Microcomputerized tomography (CT) has been applied to the development of three-dimensional (3D) models of vertebral bone and the disc space (Gruber et al. 2005).
In humans, and in the sand rat, advanced disc degenerative changes and end plate calcification (sclerosis) have been documented. Most notably, Modic et al. have contributed the major study of end plates in degenerating human discs (Modic et al. 1988). As noted above, a positive correlation was observed between the percentages of dead cells in the aging sand rat disc and end plate bone mineral density.
Micro-CT model formulation provided an additional novel, nondestructive technique to assess the end plate-disc interface and the vascular canal network within the end plate of the sand rat spine (Gruber et al. 2005). This technique revealed a solid bony surface to the end plate that was not penetrated by vasculature. Models constructed from specimens from older animals showed roughening and pitting of the end plate surface and the development of irregular margins. Figure 20.2d illustrates end plate models examined in sagittal section to make porosity of the end plate visible. Note the progressive decrease in canals within the end plate from age 2 months to 6 and 23 months of age.
20.3.2.2 Cervical Spine
Data are now available on sand rat cervical disc degeneration (Gruber et al. 2011b). Recent findings show that the same major features seen in human disc degeneration and sand rat lumbar spine disc degeneration are also present in the aging sand rat cervical discs. Scoring of digital X-rays of cervical and lumbar sites in the same animals showed that in younger animals, cervical discs exhibited a significantly greater proportion of irregular margins compared to lumbar sites (96.5 % vs. 86.2 %, respectively, p = 0.001). In older animals, cervical discs also showed a significantly greater proportion of osteophytes (4.9 % vs. 0.7 %, respectively, p < 0.0001) compared to lumbar sites. Since animal models for cervical disc degeneration are rare, the sand rat fills an important need and provides spontaneous, age-related degeneration in both lumbar and cervical spines. Figure 20.3 illustrates cervical radiologic features in a 2.1-month-old animal and compares that with degenerative changes present in a 22.7-month-old animal. In the older animal, the inset highlights the histologic features of the osteophyte marked in the digital radiograph.
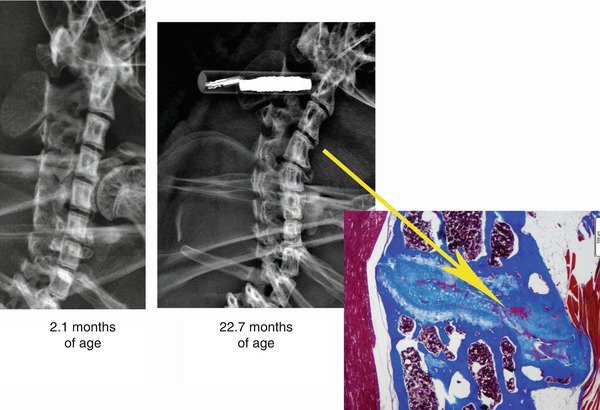
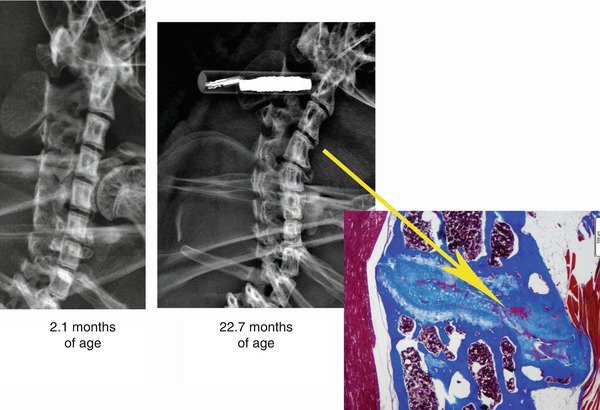
Fig. 20.3
Representative radiologic images of cervical spine features in a 2-month-old and a 22.7-month-old sand rat. An osteophyte marked in the older animal is shown in histologic section in the insert (Masson-trichrome stain; image scale = 500 μm)
20.3.3 Spin-Lock (T1r) Imaging
Studies by Regatte et al. have utilized spin-lock (T1r) imaging to study the sand rat model of disc degeneration (Regatte et al. 2004; also see Chap. 12). High-spatial-resolution images documented disc changes in both sagittal and cross-sectional planes of the disc. Figure 20.2e illustrates a sagittal plane image that shows prominent disc space narrowing (arrows) in a 4–5-month-old animal. These investigators also quantified spatial variation in the T1r relaxation times in annulus and nucleus regions. These values were in the range of 100–120 ms and 50–60 ms, respectively, at 500 Hz. Proteoglycan imaging was excellent based on exchange of protons on glycosaminoglycans with bulk water.
20.3.4 Disc Cell Death and End Plate Bone Mineral Density (BMD)
As noted above, the degenerating disc exhibits increasing end plate calcification. The bone mineral density (BMD) of cranial and caudal end plates of the sand rat lumbar vertebrae was significantly greater in older animals (Table 20.2), and caudal end plates usually had significantly greater BMD values than did cranial plates (Gruber et al. 2008a). These same specimens were used to obtain viability assays for live/dead cells within the annulus. The percentage of dead cells in the annulus correlated in a significant, positive manner with end plate BMD (p = 0.02, r = 0.347). Vertebral end plate sclerosis and disc cell viability are probably associated because diffusion of nutrients through the end plate and disc margin provides the only source of nutrition for the adult avascular disc. It is believed that end plate calcification, followed by its replacement by bone, impedes nutrient flow into the disc (Bernick et al. 1991; Bernick and Caillet 1982). In these experiments the sand rat model provided excellent information directly relevant to human disc degeneration.
Table 20.2
Analysis of the averaged end plate BMD (g/cm2) per level in the age groups
Level | Group 1: 1–3.9 months (n = 36) | Group 2: 4.0–11.9 months (n = 18) | Group 3: 12–23.9 months (n = 19) | Group 4: 24–46 months (n = 26) | P valuea |
---|---|---|---|---|---|
L1–2 | 0.064 ± 0.013 | 0.102 ± 0.016 | 0.113 ± 0.014 | 0.128 ± 0.022 | <0.001 |
L2–3 | 0.068 ± 0.015 | 0.101 ± 0.020 | 0.118 ± 0.016 | 0.134 ± 0.023 | <0.001 |
L3–4 | 0.084 ± 0.016
![]() Stay updated, free articles. Join our Telegram channel![]() Full access? Get Clinical Tree![]() ![]() ![]() |