Fig. 7.1
Static loading of the spine under a follower load configuration, resulting in a primarily axial compression of the intervertebral disc
Based on how forces are distributed from organ to tissue levels, several mechanotransduction themes have emerged from the growing body of literature. The first is that the magnitude and distribution of loads within the nucleus pulposus and annulus fibrosus govern disc cell function. The second is that load frequency and duration significantly contribute to the disc’s mechanical and mechanobiologic response. And the third is that age-related matrix changes may set off a vicious cycle of degeneration-promoting processes. In this section, we will examine the evidence that support these concepts.
Given the disc’s complex matrix structure, mechanobiologic interactions are influenced at multiple hierarchical levels. Within each disc sub-tissue, a unique combination of extracellular matrix fibers and ground substance confer different physical properties, where the extracellular matrix molecules are adapted to manage and respond to mechanical stress. Consequently, the extracellular matrix physical properties are dynamic and regulated, and create a microenvironment that presents cells with physical and biochemical cues that are important for maintenance of a healthy tissue. Cues include chemical (hypoxia, pH), biochemical (growth factors, cytokines, neurotrophins, hormones), and physical (topography, fluid flow, stiffness) factors.
7.2.1 Physiologic Interplay in Mechanical Function in the Nucleus Pulposus and Annulus Fibrosus Is Critical for Tissue Homeostasis
Intervertebral disc loading depends on an individual’s size, physical activity level, occupational demands, and degree of rest. In the human, spinal stability requires that the direction of the resultant force vectors at each vertebra passes through the centers of rotation of adjacent motion segments in the sagittal plane, a concept called follower load path (Patwardhan et al. 1999). The follower load strategy allows the spine to support static loads up to and significantly above typical physical demands (Patwardhan et al. 2000), without markedly sacrificing flexibility or range of motion (Rohlmann et al. 2001; Patwardhan et al. 2003). This suggests that during static conditions muscle activation patterns in vivo cause the disc to be primarily loaded in axial compression (Fig. 7.1), a loading mode that has been extensively studied in animal models.
In the healthy spine, the intervertebral disc responds to axial compression by nucleus pulposus pressurization facilitated by lateral annulus fibrosus constraint, which in turn generates circumferential and longitudinal annulus fibrosus tension. Preservation of this structural interrelationship is important for tissue maintenance. This is perhaps most clearly demonstrated in static compression of rodent discs. In such experiments, static loads induce fluid shifts that deplete nucleus pulposus volume, causing the annulus fibrosus to function more as a compression-bearing strut than a biaxially stretched membrane (Lotz et al. 1998). As fluid is expelled from the nucleus, the matrix and cells consolidate (Lotz 2004; Hsieh et al. 2005), ultimately leading to deregulated gene expression (Lotz et al. 1998), increased metalloproteinase (MMP) activation (Hsieh and Lotz 2003), and apoptosis (Chin et al. 1999; Lotz and Chin 2000). In both mice (Lotz et al. 1998) and rats (Iatridis et al. 1999), these cellular effects alter disc architecture and mechanics.
In contrast, beneficial effects of nucleus pulposus pressurization and annulus fibrosus tension are observed when the disc is subjected to cyclic loading. Low load magnitudes are most amenable to maintaining homeostatic stress environments in the nucleus pulposus and annulus fibrosus over extended loading durations. For example, under low cyclic loads comparable to normal activities, nucleus pulposus cells exhibit little alteration in expression of genes that would lead to matrix remodeling, regardless of loading frequency (Maclean et al. 2004). Similarly, annulus fibrosus cells undergo a low level of apoptosis and exhibit depressed expression of certain catabolic factors such as MMPs and ADAMTSs (Maclean et al. 2004; Walsh and Lotz 2004). High magnitudes of stress can be similarly accommodated for brief durations and frequencies, near 1 Hz. However, if applied at low frequency or long durations, high compression magnitudes lead to a shift in cell function and tissue morphology. Under these circumstances, nucleus pulposus cells respond via upregulation of genes associated with extracellular matrix remodeling (Maclean et al. 2004; MacLean et al. 2005; Wuertz et al. 2009), leading to decreases in disc height and mechanical stability (Ching et al. 2003, 2004). In the annulus fibrosus, lower loading frequencies also cause a shift in the balance of gene expression toward a more catabolic profile (Maclean et al. 2004).
Taken together, these studies demonstrate that physiologic load sharing between nucleus pulposus and annulus fibrosus (principally nucleus pulposus pressure and annulus fibrosus tension) promotes tissue homeostasis. This is underscored by experiments in which specific aspects of this interplay are selectively modulated. For instance, using mouse models, disc bending to induce tension and compression on opposing regions of the annulus stimulates apoptosis and altered gene expression only on the side of compression (Court et al. 2001, 2007). The restoration of annulus fibrosus tension has similarly been demonstrated to be potentially protective. Applying bending to discs that had previously been subjected to degeneration-inducing static compression was effective in preserving annulus fibrosus lamellar morphology, even though apoptosis could not be mitigated (Lotz et al. 2008). These effects can also be observed when needle stabs that reduce nucleus pulposus pressurization (and consequently annulus fibrosus tension) also trigger degenerative changes in the annulus with increased MMP-2 immunoreactivity, while those that are too small to affect disc pressurization do not (Rousseau et al. 2007; Hsieh et al. 2009; Rastogi et al. 2013).
7.2.2 Implications of Poroviscoelasticity in Nucleus Pulposus and Annulus Fibrosus Mechanobiology
Although the spine is dynamically loaded over the course of the day, trunk muscles stabilizing the spine generate resultant loads that subject discs to sustained, time-averaged compression. Due to the disc’s poroviscoelastic nature, this sustained compression results in loss of stature, contributed in large part by decreases in spine length (Koeller et al. 1984; Leatt et al. 1986). Even low-impact activities, such as gentle walking, have been shown to decrease stature significantly (Hoe et al. 1994), although more strenuous exercise, work-related activities, and high body mass exacerbate this loss (Fig. 7.2, top) (Garbutt et al. 1990; McGill et al. 1996; Leivseth and Drerup 1997; Rodacki et al. 2005). These stature changes are primarily attributed to the redistribution of fluid as the applied stresses exceed the disc’s swelling pressure (Adams and Hutton 1983; Koeller et al. 1984; Adams et al. 1990; Terahata et al. 1994; Ayotte et al. 2000; Hsieh et al. 2005; Schroeder et al. 2006).
As with other compressive load-bearing tissues such as articular cartilage, the disc’s extracellular solid–fluid interactions define its viscoelastic behavior. Interstitial fluid in the hydrated extracellular matrix serves as a hydraulic cushion that helps distribute forces and absorb shock. Sustained intervertebral disc compression consolidates the disc extracellular matrix as there is an efflux of interstitial fluid and a decrease in water content (Fig. 7.2, bottom) (Adams et al. 1990). The importance of tissue hydration in intervertebral disc mechanics has been demonstrated in a number of studies that reveal a close relationship between hydration and disc mechanical properties (Bass et al. 1997; Pflaster et al. 1997; Race et al. 2000; Han et al. 2001; Costi et al. 2002). Of note, varying water content through load, by imposition of free swelling and creep compression boundary conditions, reveals the existence of an optimal hydration point at which the effective modulus reaches a peak value (Race et al. 2000). The principles behind this have not yet been elucidated, but likely involve both physical relationships among subregions, consolidation of the tissue, and transient solid–fluid interactions in the tissue. Nevertheless, these observations highlight the importance of water content in the disc’s mechanical function.
Movement of moisture due to poroviscoelasticity is particularly relevant in nucleus pulposus mechanobiology. In human discs, the swelling properties of the nucleus pulposus are well recognized as a prominent contributor to intervertebral disc mechanics. As cut from the disc, the nucleus pulposus has a water content (WC = 1 – dry weight/wet weight) of approximately 0.85 in juvenile discs, and that decreases to approximately 0.75 in aged discs (Urban and McMullin 1988). Distribution of water also diminishes outwardly through annular lamellae, as a proportion of the nucleus pulposus water content, by 0.07, 0.11, and 0.22 from inner to mid to outer annulus fibrosus (Urban and Maroudas 1981). The marked spatial variation in fluid distribution is a unique hallmark of the intervertebral disc and plays a significant role in its function. In the nucleus pulposus, a high negative fixed charge density from concentrated localization of large aggregating proteoglycans produces increases in osmotic pressures that tend to promote tissue swelling and affects mechanically driven fluid exudation (Urban and Maroudas 1981; Urban and McMullin 1985, 1988). Studies have demonstrated that matrix alteration – particularly GAG degradation – adversely affects the poroviscoelastic behavior of nucleus pulposus tissues, intervertebral disc biomechanics, and degenerative changes in animal models (Boxberger et al. 2008).
Nucleus pulposus poroviscoelastic behavior, in turn, impacts the loading experienced by the annulus fibrosus, which itself is porous and viscoelastic. However, consistent with the similarities to ligament tissue in terms of function and microstructure, the contribution to viscoelastic properties in the annulus fibrosus is thought to be primarily flow independent. Specifically, it relies on collagen fiber bundles organized in parallel to resist tensile stresses (Broberg and von Essen 1980; Hickey and Hukins 1980; Stokes and Greenaple 1985; Cassidy et al. 1989; Holzapfel et al. 2005). The staggered discontinuous nature of the fibrillar collagen microstructure (Marchand and Ahmed 1990; Holzapfel et al. 2005) results in complex transmission of force along lamellae and has profound impact on mechanotransduction. As observed in the bovine annulus fibrosus (Bruehlmann et al. 2004a, b) and similarly in the rat tail tendon (Screen et al. 2004), tissue stretch does not uniformly propagate across the levels of collagen. Rather, straightening of collagen crimp and fibril-fibril sliding leads to nonuniformly, increasing fibril recruitment at different loads and appears to account for the majority of tissue-level deformations. These phenomena have been demonstrated both through intercellular measurements within and among collagen fibers (Bruehlmann et al. 2004a, b; Screen et al. 2004) and by measuring the deformation of photobleached lines across labeled collagen fibers (Bruehlmann et al. 2004b). The mechanisms and kinetics of recovery, whether passive or actively cell-mediated, are not yet clear. Thus, not only is cell stretch a mechanism of stimulation during annulus fibrosus deformation, but shear stress likely also serves as a robust determinant of cell function.
Poroviscoelastic interactions between the nucleus pulposus and annulus fibrosus cause nucleus pressurization and annulus tension to be time dependent, modulated by the nucleus pulposus hydration state and degree of annulus fibrosus stress relaxation (Fig. 7.3). Thus, for any spinal load applied over a finite duration, the micromechanical loading regime imposed on disc cells is defined both by the current stress to the motion segment and by the history of earlier experienced applied stresses. One demonstration of this dependence on the time history of loading is illustrated in the study of Hwang et al. (2012). This study showed that in rat discs, the effectiveness of the nucleus pulposus to pressurize varied according to the specific sequence of loading events and not just by the applied endpoint stress. Meanwhile, disc height was determined only by the endpoint stress and independent of temporal path of axial loads. This load history dependence of the internal pressure and shear could then have an impact on cellular mechanotransduction and differentiation.
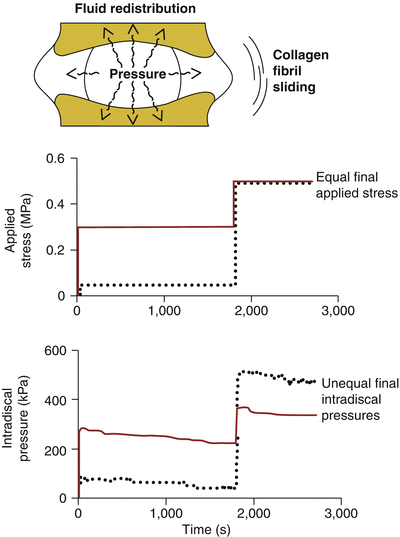
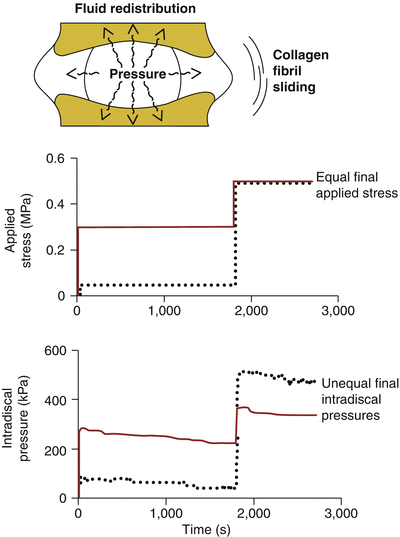
Fig. 7.3
Poroviscoelastic phenomena affect the internal mechanics of the disc (top). Graphs show that two temporal paths (solid and dotted curves) of applied stress (middle) lead to markedly different intradiscal pressures generated (bottom). Despite equivalent applied stresses at the end, the path with a higher intermediate load exhibited inferior final pressures (solid, bottom) than that with the lower intermediate load (dotted, bottom) (Adapted from Hwang et al. 2012)
As a corollary, there is also the implicit potential for restoring the homeostatic combination of nucleus pulposus pressure and annulus fibrosus shear. Introducing detours in the temporal path of spinal loading could have restorative effects on intervertebral disc hydration. Results from Gabai et al. (2007) using rat discs suggest that interspersing repeated axial compressive stresses with short, low-magnitude tensional loading allows intervertebral discs to preserve the pressure-shear balance between nucleus pulposus and annulus fibrosus. Thus, exploiting this phenomenon could be one strategy toward preventative maintenance.
While very pronounced in small animal discs, such load history effects in adult human intervertebral discs remain uncharacterized. It is possible that the temporal sequence of loading is more significant during early growth in juvenile discs and that the decreased baseline tissue hydration and calcification of end plates in aged discs reduce the impact of load history. If this is the case, the argument could be made for greater focus on spinal health in younger populations.
There are some parallels between the time load history effects and age-related changes in the intervertebral disc that are interesting to note. Similar to the expression of fluid out of the intervertebral disc with sustained compression, the aging disc possesses diminished swelling pressures and impaired capability to retain water. As such, the aged disc may represent a state in which an adverse pressure-shear microenvironment is permanently maintained, resulting in a vicious cycle of matrix catabolism and abnormal matrix synthesis. Evidence in the literature demonstrating decreases in intradiscal pressure generation and increasing numbers of spikes in the stress profile with age and degeneration supports the notion that pressure and shear remain in a state of imbalance in the adult disc (Adams et al. 1996).
7.3 Cellular and Molecular Levels
7.3.1 Studies of Disc Cell Mechanical Responses In Vitro
Cells sense and respond to mechanical cues via a plethora of mechanisms that typically operate in four steps: mechanical coupling, mechanotransduction, signal transmission, and cell response. Mechanocoupling is facilitated by cellular load transducers that include integrins, ion channels, G protein-coupled receptors, and tyrosine kinase receptors (Fig. 7.4). These lead to cell-ECM adhesion assemblies that are sensitive to reciprocal tension between the cell and matrix.
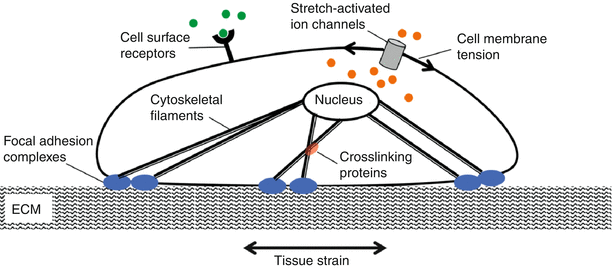
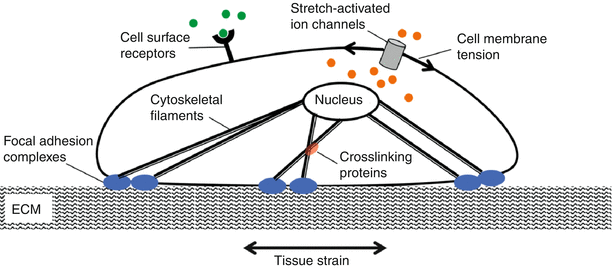
Fig. 7.4
Cells possess several mechanisms by which they sense mechanical signals. These include the stretch-activated ion channels and cytoskeletal elements that are connected to matrix via focal adhesions
Integrins are a class of cell membrane proteins that mediate adhesion of cells to substrates (Fig. 7.5). They consist of two transmembrane glycoprotein subunits with the extracellular domains interacting to form a functional heterodimer. Integrins functionally serve to connect the extracellular matrix with the cytoskeleton and have the capacity to bind collagen and fibronectin among other matrix constituents. This integrin/matrix bond may be influenced by tensile loading and matrix stiffness, with higher forces and stiffer matrices leading to enhanced attachment (Paszek et al. 2005). These types of load-dependent interactions are called catch bonds (Friedland et al. 2009). Mechanical reinforcement of “catch bonds” between the cell and matrix mediates integrin assembly and development of clusters to form focal adhesions. Focal adhesions elicit a reciprocal actomyosin-mediated cell contractility that is essential to amplify the mechanoresponse (Sniadecki and Chen 2007; Na et al. 2008; Wang et al. 2008). The affinity of integrins for extracellular matrix proteins can be modified by tissue deformation and molecular conformation force-dependent unfolding (such as with fibronectin) that can expose binding sites (Vogel and Baneyx 2003).
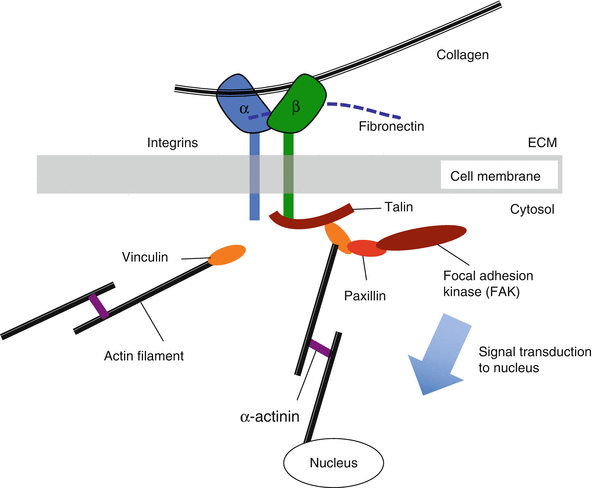
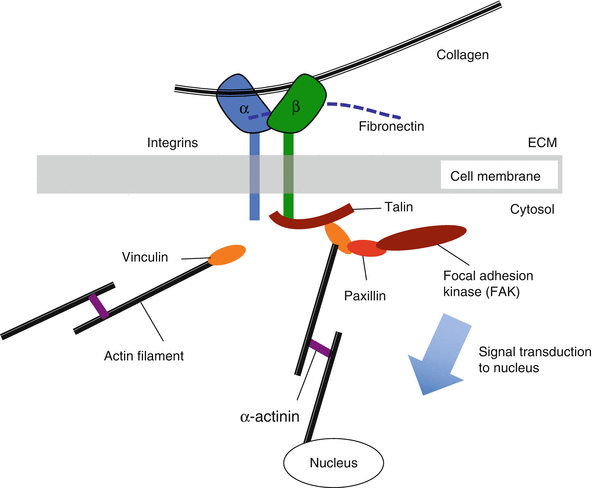
Fig. 7.5
Schematic of mechanism by which integrins interact with adhesion plaque proteins and cytoskeleton to activate specific downstream signaling
Disc cells express integrin receptors that vary by region and degree of degeneration. These include fibronectin-binding integrins (α5β1 and αvβ3), collagen-binding integrins (α1β1, α2β1, and αvβ1), and laminin-binding integrins (α6β1 and α6β4) (Nettles et al. 2004; Xia and Zhu 2008; Chen et al. 2009); laminin-binding integrins are more prevalent in the nucleus (Gilchrist et al. 2007; Chen et al. 2009). Within the nucleus, fibronectin fragments are known to accumulate with disc degeneration (Oegema et al. 2000) and trigger upregulation of α5β1 integrin expression and ERK signaling of catabolic processes (Xia and Zhu 2011).
Mechanotransduction occurs when extracellular matrix forces are coupled to the cytoskeleton and induce conformational changes of intracellular proteins that alter substrate availability leading to phosphorylation (Doyle and Yamada 2010; del Rio et al. 2009). Mechanosensing can also occur via mechanically gated calcium channels. Transmembrane ion channels influence the cell’s lipid bilayer organization and tension (Fig. 7.6). When membrane tension increases (as observed during osmotic swelling), it can alter the channel cross section and protein configuration, leading to channel opening, even in the absence of direct activation by a specific chemical ligand (Janmey and Kinnunen 2006). Calcium modulates cell activity, growth and differentiation, motility, intercellular coupling, and apoptosis. Several studies demonstrate that disc cells can respond to mechanical loading via activation of calcium channels that cause intracellular calcium transients. This type of calcium signaling has been shown to be important in regulatory volume fluxes that are observed after hypo-osmotic or fluid-induced shear stress (Elfervig et al. 2001; Pritchard and Guilak 2004). Calcium transients can lead to F-actin remodeling that facilitates reestablishment of cell volume after an osmotic challenge.
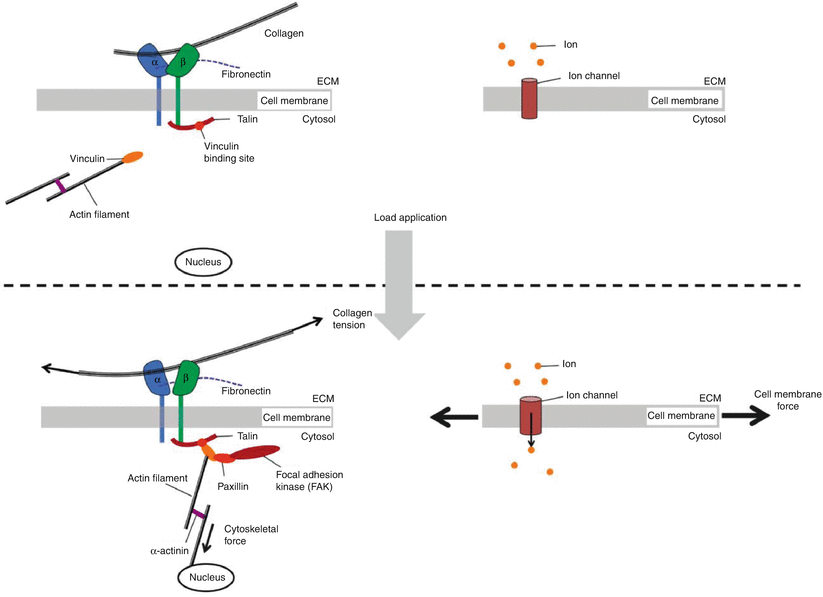
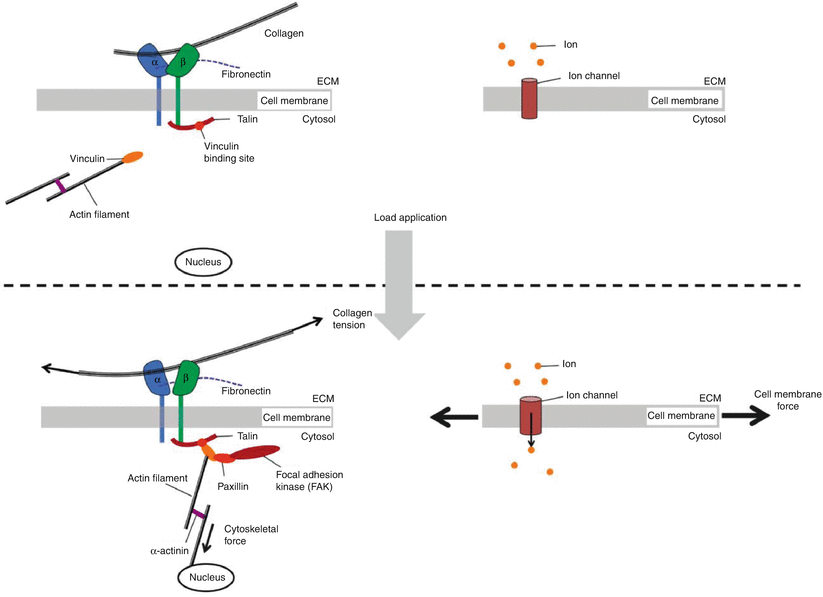
Fig. 7.6
Mechanotransduction can be viewed as a series of rapid switch-like events, activated in response to applications of force
Signals are propagated deep within the cell by the stiff cytoskeletal filaments (microfilaments (F-actin), intermediate filaments (vimentin, cytokeratin), and microtubules (tubulin)) that link the cell nucleus to the extracellular matrix via focal adhesion complexes and hemidesmosomes (Alenghat and Ingber 2002). Because cells contain a continuum of cytoskeletal elements, they display an integrated mechanical behavior, whose properties depend on the composition and organization of the cytoskeleton, and extracellular matrix interactions via transmembrane proteins, cellular proteins, subcellular structures, and intracellular fluid volume and composition (Ingber 2003). Predictably, the mechanical properties of disc cells display zonal variations in cytoskeletal composition. While disc cells demonstrate an overall viscoelastic behavior, nucleus pulposus cells are three times stiffer and more viscous than annulus cells (Guilak et al. 1999).
Microtubules form an extensive mesh throughout the cytoplasm in both nucleus and annulus cells (Li et al. 2008). Likewise, vimentin filaments are densely distributed within the nucleus and annulus cells and extend into annular cell processes (Johnson and Roberts 2003; Li et al. 2008). Nucleus pulposus cells are also characterized by the presence of cytokeratin intermediate filaments. Pronounced differences exist in F-actin distribution between the nucleus and annulus cells. In the nucleus, F-actin is localized to punctate regions of the cell membrane, while in the annulus along with vimentin, it is more pronounced and extends into cell processes (Errington et al. 1998; Bruehlmann et al. 2002; Li et al. 2008).
Mechanoresponses involve downstream intracellular signaling and transcription networks. Intracellular signal transduction can occur via the cytoskeleton, small molecules (second messengers Ca2+, 1,4,5-triphosphate (IP3), cAMP), protein kinases (focal adhesion kinase, cSrc, protein kinase C, mitogen-activated protein kinase), and transcription factors (c-fos, c-jun, c-myc, NF-kB).
Cellular response pathways function over different timescales that can define frequency-dependent cell behaviors (Fig. 7.7). For example, force can cause an immediate cell response (hundreds of milliseconds) through activation of ion channels or conformational changes in the cytoskeleton. In contrast, other signaling pathways require polymerization of cytoskeletal stress fibers and alterations of intracellular protein networks and act over minutes. Still others may take hours or days as they act by causing changes in gene expression that influence cytoskeletal or adhesion proteins and ultimately force-transmission pathways. Consequently, these time-dependent behaviors lead to frequency-dependent signaling, where cells function as band-pass filters (Hoffman et al. 2011). To be stimulated, a particular signaling pathway needs to match the timescale of the applied forces.
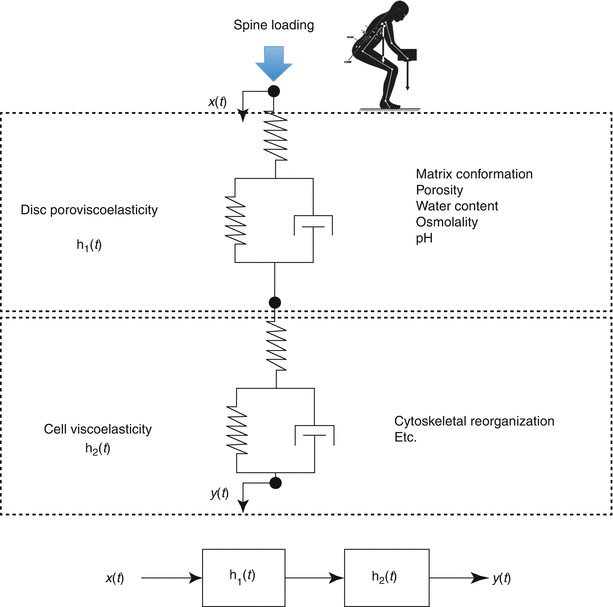
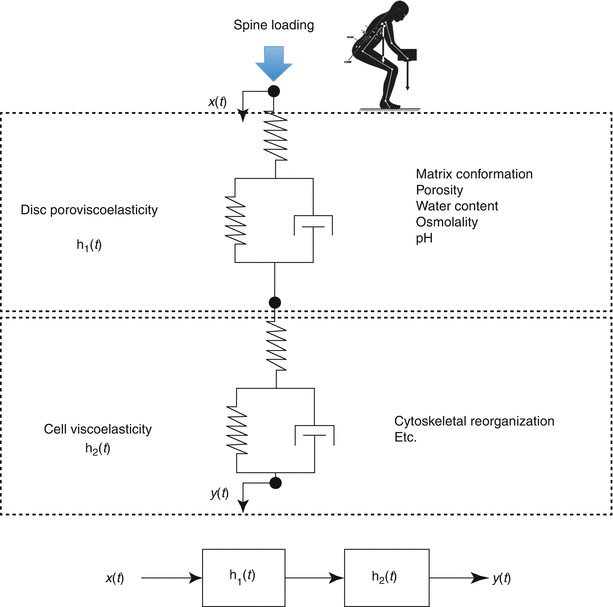
Fig. 7.7
A conceptual scheme of the multiscale, multifactorial influences on disc cell mechanobiology. The time-dependent cellular response (y(t)) resulting from spinal loading (x(t)) is dependent on dynamic tissue and cellular processes that may be quantified by transfer functions h1(t) and h2(t), respectively
7.3.2 Disc Cell Responses to Mechanical Perturbation
Disc cell phenotype varies between the annulus and nucleus, and accordingly, these cells are considered to have distinct repertoires of load-induced behaviors. These differences are likely due to spatially dependent adaptation to hydrostatic pressure (nucleus) and stretch (annulus) that are developed during spinal loading. To facilitate systematic study of cell mechanoresponsiveness, a number of in vitro test systems have been developed (Brown 2000). These allow the controlled application of known inputs (either stress or strain) so that dose–response functions can be established. Independent variables in these in vitro bioreactor studies include type, magnitude, frequency, and duration of mechanical stimulation.
7.3.3 Disc Cell Response to Pressure
The healthy disc nucleus is largely water and, consequently, is subjected to hydrostatic pressure during spinal loading. For that reason, both monolayer (2D) and hydrogel (3D) culture systems have been used to subject nucleus pulposus cells to hydrostatic pressure or compression. Nucleus cells are generally considered chondrocyte-like, while annulus fibrosus cells are fibroblastic. Accordingly, nucleus cells prefer the environment of inert hydrogels such as alginate or agarose that support a spherical configuration conducive to the assumption of a stable chondrocyte-like phenotype (Chen et al. 2002). In contrast, in monolayer culture, nucleus cells dedifferentiate as cell spreading leads to significant downregulation of Col2 gene expression and a change to a fibrotic phenotype with increased growth kinetics (Horner et al. 2002; Kluba et al. 2005; Rastogi et al. 2009; Wang et al. 2011). Conversely, annulus cells do less well in 3D culture in terms of cell morphology and survival (Horner et al. 2002).
Interpretation of published studies on disc cell mechanoresponsiveness is confounded by the fact that disc cells are isolated from many different tissue sources. These include human surgical samples and small and large animals. While human cells may be desirable to study disease pathogenesis, they are logistically difficult to acquire without close affiliation to high-volume surgical centers. Also, studies with human cells are complicated by individual-to-individual variability and degeneration-related phenotypic shifts (Kluba et al. 2005; Gruber et al. 2007). These factors will lead to experiment-to-experiment variability and require larger sample sizes for identifying statistically significant effects. In comparison, animal tissues are more readily available, but can be limited by the presence of persistent notochordal cells, which are lost in adolescence in humans but are maintained throughout life in mice, rats, rabbits, pigs, cats, and non-chondrodystrophic dogs (Risbud and Shapiro 2011; Hunter et al. 2003). The nucleus pulposus of several species has been observed to convert from notochordal to chondrocyte-like cells with age in a similar fashion to humans. These include cattle, horses, sheep, and chondrodystrophic dogs (beagles) (Miyazaki et al. 2009). Not surprisingly therefore, interspecies differences have been reported for disc cell responsiveness (Minogue et al. 2010; Miyazaki et al. 2009; Sakai et al. 2009), particularly when distinguishing notochordal from non-notochordal nucleus tissues (Miyazaki et al. 2009). Bovine tails may represent the best compromise for in vitro investigations, as they have a non-notochordal nucleus and are easy and inexpensive to procure in large numbers. For a full discussion of animal preferences in intervertebral disc research, see Chap. 18.
Hydrostatic pressure chambers are most commonly used to study disc cell responsiveness to compression loading (Hutton et al. 2001; Liu et al. 2001; Kasra et al. 2003; Wenger et al. 2005; Reza and Nicoll 2008). Alternatively, some use confined or unconfined compression of 3D gel/cell constructs. To simplify the latter tests, loading platens are often operated in displacement control, so that compressive strain, rather than compressive stress, becomes the independent test variable (Chen et al. 2004; Korecki et al. 2009; Fernando et al. 2011; Salvatierra et al. 2011; Wang et al. 2011). Note that, in Chap. 22, further details are provided on choices of bioreactors for disc research.
In vitro loading parameters are typically based on values obtained from human disc studies. For example, studies of human subjects suggest that due to the inherent resonant frequency of the torso, certain loading frequencies may be deleterious (between 4 and 6 Hz; Wilder and Pope 1996; Kumar et al. 1999). Due to upright posture, static or low-frequency (1 Hz) pressures from gravity loading and daily living activities are also of interest given the recognized differences in disc degeneration in humans when compared to quadrupeds (Lotz 2004). Accordingly, in vitro studies explore cell responses over a broad range of loading frequencies, from static to 20 Hz (Table 7.1).
Table 7.1
Experimental in vitro studies of pressure on disc cell function
Cell type | Cell source | Scaffold | Load | Frequency (Hz) | Duration | Reference |
---|---|---|---|---|---|---|
AF | Porcine | Alginate | 1–3 MPa | 0.5 | 3 h | Wenger et al. (2005) |
NP | Rats | Alginate | 10 kPa, 20 % strain | 0.5 | 1 h | Wang et al. (2011) |
AF, NP | Porcine | Agarose | 15 % strain | 2 | 4 h | Salvatierra et al. (2011) |
IAF, OAF | Bovine | PGLA | 5 MPa | 0.5 | 4 h/day, 3–14 days | Reza and Nicoll (2008) |
NP | Human, bovine | Col 1 | 0.25 and 2.5 MPa | 0.1 | 1 h, 24 h | Neidlinger-Wilke et al. (2009) |
NP | Human, bovine | Col 1 | 0.25, 2.5 MPa | 0.1 | 24 h | Neidlinger-Wilke et al. (2006) |
AF, NP | Human | Tissues | 0.1, 0.3, 3 MPa | Static | 2 h | Liu et al. (2001) |
AF, NP | Human | Alginate | 0.35–0.95 MPa | 1 | 2 h | Le Maitre et al. (2009) |
AF, NP | Porcine, bovine | Alginate | 2–12 % strain | 3 | 2 h/day, 7 days | Korecki et al. (2009) |
AF, NP | Rabbit | Alginate (NP) | 0–3 MPa | 1–20 | 30 min/day, 3 days | Kasra et al. (2003) |
AF, NP | Porcine | Alginate | 1 MPa | 1, 3, 5, 8, 10 | 30 min/day, 3 days | Kasra et al. (2006) |
AF, NP | Canine | Alginate | 0.35, 0.1 MPa | Static | 9 days | Hutton et al. (2001) |
IAF, OAF, NP | Human | Tissue | 0.3, 3.0 MPa | Static | 2 h | Handa et al. (1997) |
NP | Rabbit | Alginate | 0.7, 2, 4 MPa
![]() Stay updated, free articles. Join our Telegram channel![]() Full access? Get Clinical Tree![]() ![]() ![]() |