Comparisons of site-specific loading in young jockeys (mean age 19 years) with age-matched controls showed lower bone strength at the tibia. However, at other occupationally specific sites such as the radius, jockeys showed greater bone strength and trabecular density, independent of bone size (Wyner, 2010).
Specificity of gymnastics in upper and lower limbs
As a widely practiced activity by very young athletes, gymnastics has been studied extensively. Many gymnastics skills have been analyzed to determine the specific impact loading exerted on the skeleton. Ground reaction forces to both the upper and lower extremity have previously been recorded. Often, advanced level skills can generate forces of up to 13–14 times body weight on young highly trained gymnasts. Few intermediate skills have been assessed, with forces varying from 2 to 4 times body weight for the wrists and 10 times bodyweight for the ankles. Estimates of national and international gymnasts’ upper and lower body ground reaction forces confirmed higher forces in the more experienced, skilled gymnasts.
Video analysis of the frequency of gymnastic-specific movements has been also used to estimate gymnastics loading. Gymnastic-specific movements including static, swing, and impact movements have been used to quantify the frequency of gymnastics loading during different phases of training, for male international level gymnasts. In this study (Burt et al., 2010), gymnastics training was associated with, on average, 102 and 217 impacts per session on the upper and lower extremities, respectively with peak magnitudes of 4 and 10 times body weight.
One can point out that the wrist is subjected to forces in gymnastics that can exceed two to four times body weight. Therefore, the regular use of the upper extremities to support body weight subjects the wrist joint to recurrent impacts and loading. Wrist pain is common among artistic gymnasts of both sexes. Estimates of wrist pain in these participants range from 46 to 79% (Mandelbaum et al., 1989). In addition, more than 50% of young, beginning to mid-level, gymnasts also experience wrist pain. Cross-sectional surveys indicate that approximately 45% of gymnasts report pain of at least 6 months’ duration.
There may be many possible causes of wrist pain in young gymnasts including dorsal wrist impingement, triangular fibrocartilage complex, and ulnar impaction syndrome (DiFiori et al., 2006). However, because nearly all gymnasts enter the sport at a young age, the growth plates of the wrist are also potential sites for injury. Roy et al. (1985) described a stress reaction in a series of young gymnasts (Figure 4.2). Since then, there have been multiple case reports and case series describing stress injury to the distal radial physis. The cause of physeal injury in this setting may be a compromise of the metaphyseal and/or epiphyseal blood supply and may, infrequently, result in premature partial or complete closure of the epiphysis (DiFiori, 2006). The reports of distal radial physeal arrest are consistent with experimental studies in which prolonged intensive physical loading is associated with inhibition of linear bone growth. These findings suggest repetitive physical loading in excess of tolerance limits as a principle etiological factor in distal radius physeal injury and growth disruption. Unfortunately, other potential etiological factors such as nutrition, technique, and equipment have not been well studied.
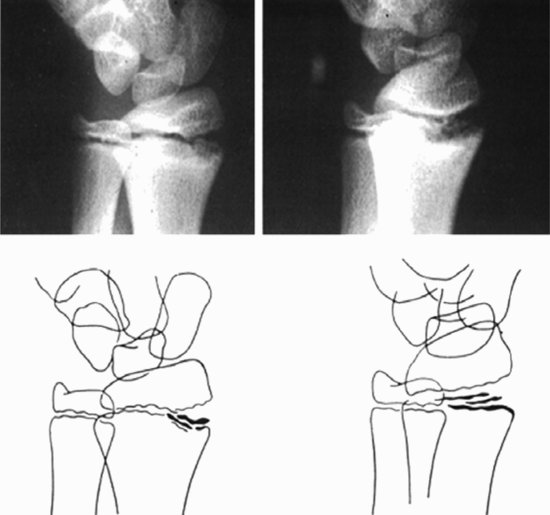
Figure 4.2 Radiographs and line drawings of the wrist of a symptomatic young gymnast. Changes of the distal radial epiphysis include widening of the radial margin, fragmentation, cystic changes and irregularity of the metaphyseal margin, and a beaked effect of the distal aspect of the epiphysis. (Source: Roy S, Caine D, Singer KM (1985) with permission from SAGE Publications.)
In summary, repetitive loading of the wrist joint in young gymnasts is frequently associated with wrist pain, and injury to the distal radial physis occurs in some individuals. However, the relationship between these events and the development of positive ulnar variance is less clear.
Skeletal responses to mechanical loading at pre-, peri-, and post-pubertal stages
The osteogenic benefits obtained from exercise during growth are maturity- and sex-dependent. Exercise interventions seem to be most effective when initiated during pre- or early puberty. It is thought that exercise may preferentially affect the surface of bone that is undergoing apposition during growth. Before puberty, the skeleton reveals the capacity of responding to loading by adding more bone on the periosteal surface than would normally occur through growth-induced periosteal apposition.
Several studies have suggested that the skeletal response to activity varies by pubertal stage. A 6-year longitudinal study (Saskatchewan bone mineral accrual study; Bailey et al., 1999) showed that active children gained more bone during the 2 years surrounding peak bone velocity than inactive children (from 10 to 40% depending on the skeletal site). In this study the accrual rates for the different maturation stages were not assessed.
A cross-sectional Finnish study analyzed the relationship between maturity and BMD in child and adolescent female racquet sport players and maturity-matched controls (Haapasalo et al., 1998). In this study, the side-to-side BMD differences were measured at the proximal humerus, humeral shaft, and distal radius. The differences were significantly greater in players than controls at Tanner stages 3, 4, and 5. There were no differences between players and controls at Tanner stage 1, while the girls differed at the humeral shaft for Tanner stage 2. A potential correlation between training volume and intensity, and maturation stages could bias such observations, but it seems that after stage 3 the association of training and bone gain suggest a synergistic effect of pubertal hormone activity and mechanical loading in explaining the bone accretion.
Knowing the stage of development at which bone is most responsive to mechanical constraints offers an attractive possibility to enhance bone mass to benefit children by means of a physical activity program.
Ironically, as age increases in the majority of children, and girls in particular, physical activity decreases. Intuitively, establishing good physical activity patterns prior to puberty appears appropriate. Promoting sports such as gymnastics with great attraction for girls and well-established musculoskeletal benefits would seem to be a developmentally appropriate strategy.
Differential effects of gymnastics on bone mass, density, geometry, architecture, and bone strength index
Gymnastics training is unique in generating high mechanical loading to the skeleton of very young children. Most of the studies have focused on competitive female participants. Recently, studies have extended to recreational gymnasts, using longitudinal follow-up designs. In these studies, a large range of participants has been analyzed, from 4 to 17 years old. Indeed, this age span includes peripuberty in which bone is highly responsive to loading stimulations. As such, gymnastics provides an excellent model for assessing the effects of weight-bearing physical activity on bone mineral development.
Bone mineral density
To date, there is evidence for an osteogenic effect of gymnastic training, specifically via bone mass and density.
From cross-sectional studies, girls training regularly in gymnastics for 2 or more years have displayed a higher BMD than their untrained counterparts at specific skeletal sites. Prepubertal girls who participated in elite level gymnastics had greater BMD at the mid-radius, distal radius, lumbar spine, femoral neck, and Ward’s triangle than age-matched peers. In contrast, prepubertal female swimmers failed to show differences in BMD values when compared with age-matched nonswimmers (Courteix et al., 1998; Figure 4.3). These differences may be attributable to the intermittent high-impact ground reaction forces, such as jumping and tumbling that are involved in gymnastics, as opposed to the generation of muscular forces in a low-gravity environment such as swimming.
Figure 4.3 BMD values for gymnasts and swimmers, expressed as a percentage of control values. *p < 0.05; **p < 0.01; ***p < 0.001; NS, difference between groups is not statistically significant. (Source: Courteix et al. (1998) with permission from Springer Science + Business Media.)
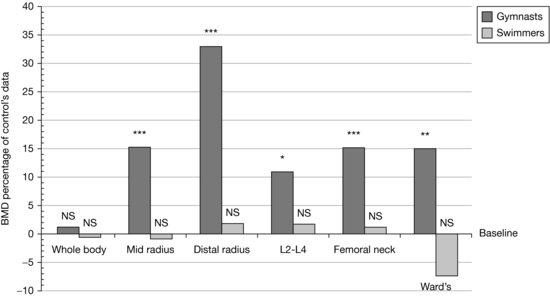
Some studies have assessed the link between the volume (duration and intensity) of training and the magnitude of improvement in bone mass gain. Results indicate that the higher the volume of practice, the greater the enhancement of bone mass.
Longitudinal studies have reported that areal BMD increased 30–85% more rapidly in young female gymnasts than in sedentary children. One study compared the bone mineral accrual of high-level versus recreational participation in gymnastics by 4–10-year-old girls (Erlandson et al., 2011b). The findings suggest that recreational and precompetitive gymnastics participation is associated with greater BMC. This study emphasizes the ability of low-level gymnastics participation to positively influence bone accretion. Therefore, participation in gymnastics even through school physical education programs should be promoted for all children.
The association of sports participation to longitudinal changes in BMD in collegiate female athletes aged 18–29 years was explored in gymnasts, runners, swimmers, and controls, in two cohorts followed-up for either an 8-month or a 12-month period (Taaffe et al., 1997). These results pointed out the highest BMD values in gymnasts. During follow-up, the gymnasts were the only participants to exhibit positive gains at both the lumbar spine and femoral neck.
Beyond the use of dual energy X-ray absorptiometry (DXA) in investigating the bone density of skeleton, recent investigations have also used more precise methods to explore the changes of shape, size, and geometry within bone compartments.
Although BMD is one of the most important factors contributing to bone strength, it cannot explain alone how the bone tissue organization evolves under the constraints. Other factors including geometry and microarchitecture are involved in explaining bone strength.
Geometry
The mechanical behavior of bone not only depends on its material properties but also largely depends on the spatial distribution of bone material within the given structure. Geometric factors such as external diameter and cortical thickness can significantly influence bone strength. The outer diameter of long bone can, for example, predict up to 55% of the variation in bone strength.
Most bone geometric research in retired gymnasts have been performed at the forearm, with results often showing greater indices of total bone size and strength in gymnasts than controls.
In a recent cross-sectional study, the geometry of 173 gymnast and control participants, 8–17 years old, was analyzed using the hip structural analysis technique (Dowthwaite et al., 2012). Maturity, assessed by the stages described by Tanner, was taken into account in this study. Maturity-specific comparisons suggested site-specific skeletal adaptation to loading during growth, with greater advantages at the radius than the proximal femur. At the radius, gymnasts’ advantages included greater bone width, cortical cross-sectional area (CSA), and cortical thickness; in contrast, at the femoral neck, gymnasts’ bone tissue CSA and cortical thickness were greater, but bone width was narrower than in nongymnasts.
Although hip structural analysis provides interesting additional data, it remains limited by its two-dimensional techniques. Peripheral quantitative computed tomography (pQCT) provides three-dimensional (3D) analyses in regions of interest to bone loading such as the tibia and radius. Devices such as pQCT are included among instruments capable of providing more informative 3D analyses of bone geometry at the “macroarchitectural” level.
Macroarchitecture: bone strength
Bone geometry can be an important component of the quantitative assessment of bone. Understanding bone strength at the macroarchitectural level involves the characteristics of shape and structural integrity, particularly from data acquired for cortical bone.
It is also possible to acquire microarchitectural characteristics to improve the understanding of bone geometry at a more precise level and include information about trabecular volume, trabecular spacing, and connectivity. Microarchitectural analyses will be discussed later in this chapter.
Devices such as the pQCT provide macrostructural characteristics such as cortical area, CSA, and density. However, one of the most interesting components of bone geometry is bone strength. Essentially, bone strength is the product of bone structure (size and shape) and material properties (density). Using estimates obtained from a pQCT device, bone strength at a specific site is calculated by first dividing the cross-sectional moment of inertia by 0.5 of the subperiosteal width and then multiplying the quotient by the cortical density.
In a comparison of well-trained adolescent female athletes, gymnasts showed 60% and 53% greater bone strength at the distal and proximal tibia, respectively, than their nonactive peers (Greene et al., 2012). Independent of bone size, bone strength differences can be found. Weight-bearing bones in small people can continue to develop strength by laying down cortical bone on the inside surface and/or through improving cortical density.
For example, when tibial bone characteristics of young apprentice jockeys were compared with age-matched peers, the apprentice jockeys showed lower bone strength at the tibia than controls. However, greater bone strength was apparent at the radius of the jockeys than the controls. Site-specific loading adaptations are therefore well supported by macroarchitectural estimates of bone strength.
Longitudinal results from recreational and competitive gymnasts who were tracked for 6 months just prior to, or in the early stages of puberty, showed the greatest bone strength gains in the radius of the gymnasts who were most exposed to gymnastics over this period of time. From the longitudinal results, we can also improve our understanding of how dose-responsive musculoskeletal adaptations can occur from participating in sports such as gymnastics.
Measures of bone strength have also been used in combination with body weight and forearm length to estimate fracture risk in the radius and ulna of gymnasts. Greater bone strength to weight ratios were found in 6–11-year-old gymnasts than non-gymnasts at the proximal ulna than radius (Burt et al., 2011). This has also been shown in retired elite gymnasts. The traditional measurement site of the radius may therefore be underestimating the magnitude of exercise-induced skeletal adaptations that can be associated with participating in sports such as gymnastics.
Microarchitecture
Access to parameters of bone quality has improved in recent years with the refinement of bone imaging techniques. Using high-resolution pQCT (HR-pQCT) or magnetic resonance imaging, 3D images of the radius and tibia with a high resolution can be readily achieved. This provides in vivo access to parameters such as percentage of bone volume (BV/TV), cortical thickness (Ct.Th), trabecular number (Tb.N), trabecular thickness (Tb.Th), and trabecular separation (Tb.Sp).
Trabecular bone microarchitecture is an important skeletal feature that identifies individuals with fracture risk as well as, or better than, BMD. The unique study (Modlesky et al., 2008) that investigated the trabecular bone microarchitecture, such as apparent trabecular bone volume to total volume (appBV/TV), trabecular number (appTb.N [mm−1]), trabecular thickness (appTb.Th [mm]), and trabecular separation (appTb.Sp [mm]) using magnetic resonance imaging in young female artistic gymnasts exhibit higher appBV/TV (14%) and appTb.N and lower appTb.Sp (14%) than controls (Figure 4.4).
Figure 4.4 High-resolution magnetic resonance images of the proximal tibia of a female collegiate artistic gymnast (A) and a female control (B). There is a greater amount of trabecular bone (black) and less marrow (white) in the gymnast’s proximal tibia, which is highlighted by the magnified subregions presented at the top right of each image. (Source: Modlesky et al. (2008) with permission from Springer Science + Business Media.)
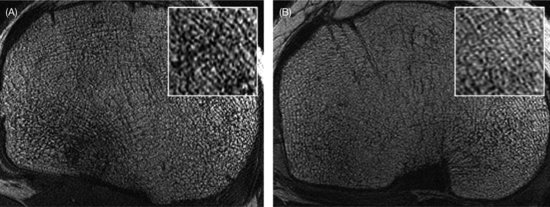
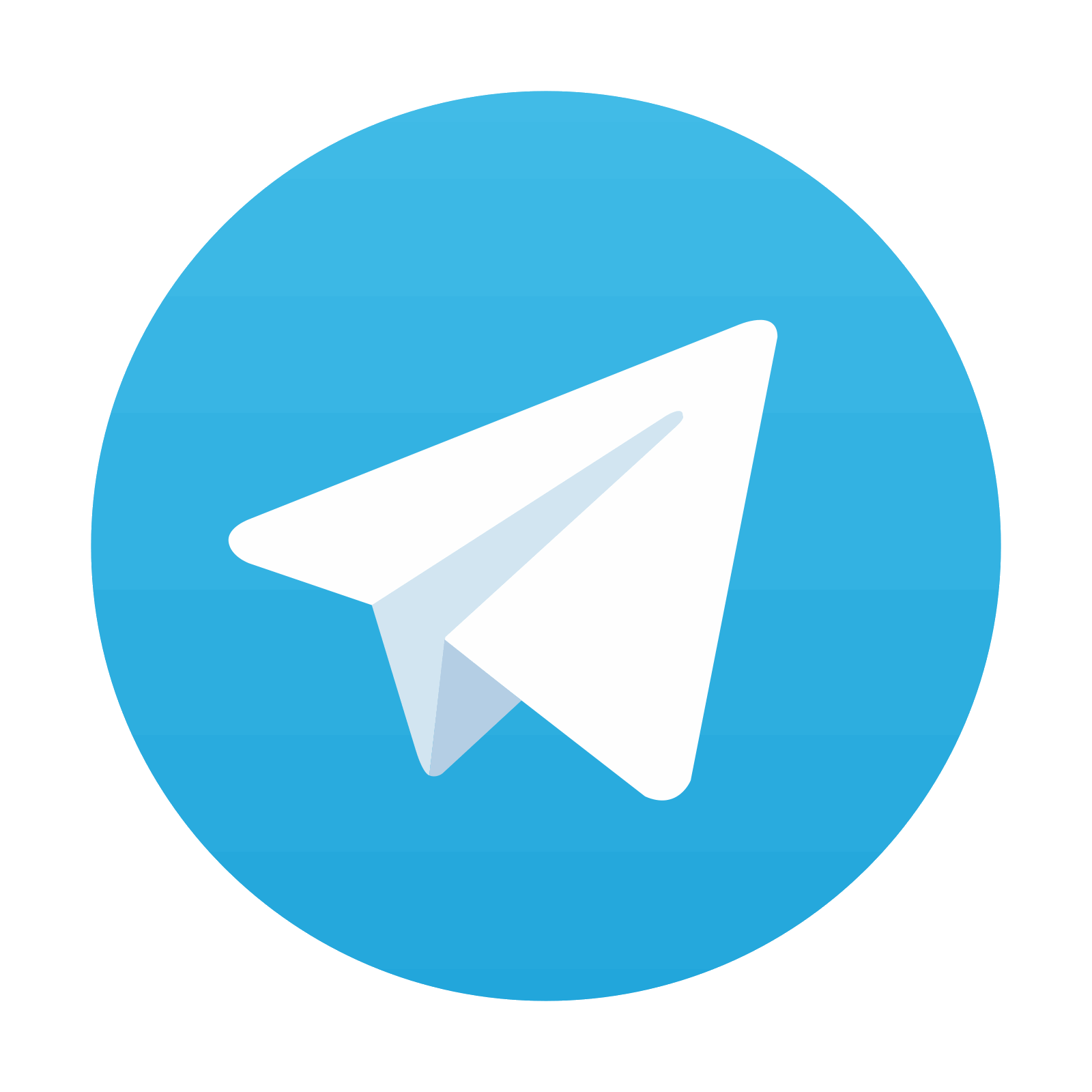