Fig. 18.1
Rotator cuff muscle anatomy. Supraspinatus muscle can be seen in the superior view, infraspinatus and teres minor muscles in the posterior view, and subscapularis in the anterior view. All muscles exhibit a multipennate muscle fiber arrangement
18.7 Experimental Determination of Skeletal Muscle Architecture
Quantitative studies of muscle architecture were pioneered by Gans and his colleagues [23, 24], who developed a precise methodology for defining muscle architecture based on microdissection of whole muscles. The parameters usually included in an architectural analysis are muscle length, fiber or fascicle length, pennation angle (i.e., the fiber angle relative to the axis of force generation), and physiological cross-sectional area (PCSA). Typically, muscles are chemically fixed in formalin to maintain fiber integrity during dissection.
Pennation angle (θ) is measured by determining the average angle of the fibers relative to the axis of force generation. Usually, only the pennation angle of fibers on the superficial muscle surface is measured, although pennation angles may vary from superficial to deep and also from proximal to distal. Although more sophisticated methods could be developed for measurement of pennation angle (e.g., diffusion tensor imaging), it is doubtful they would provide a great deal more insight into muscle function because variations in pennation angle do not dramatically affect function [23].
Muscle length is defined as “the distance from the origin of the most proximal muscle fibers to the insertion of the most distal fibers” [25]. Fiber length represents the number of sarcomeres in series, and experimental evidence suggests that muscle fiber length is proportional to fiber contraction velocity [24, 26]. Muscle length and fiber length are not always the same because there is a variable degree of “stagger” seen in muscle fibers as they arise from and insert onto tendon plates (Fig. 18.1). Muscle fiber length can only be determined by microdissection of individual fibers from fixed tissues or by laborious identification of fibers by glycogen depletion followed by serial sections along the length of the muscle [27]. Unless investigators are explicit when they refer to muscle fiber length, they are probably referring to muscle fiber bundle length (also known as fascicle length) because it is extremely difficult to isolate intact fibers that run from origin to insertion, especially in mammalian tissue [27, 28]. In muscle architecture studies, bundles consisting of 5–50 fibers are typically used to estimate fiber length, which may be reported as either fiber length or fascicle length.
The final and crucial experimental step required to perform architectural analysis of a whole muscle is to measure the sarcomere length within the isolated fibers. This is necessary to compensate for differences in muscle length that occur during fixation. In other words, to conclude that a muscle has “long fibers,” one must ensure that it was not merely fixed in highly stretched position corresponding to a long sarcomere length. Similarly, muscles with “short fibers” must be further investigated to ensure that they were not simply fixed at a short sarcomere length. To permit such conclusions, fiber length mea-surements should be normalized to a constant sarcomere length. Fiber (or fascicle) lengths are usually normalized to the sarcomere length at which the sarcomere generates maximum force. This normalized fiber length is referred to as optimal fiber (or fascicle) length and provides a reference value that can be related back to the physiologic length if the relationship between muscle length and joint position is noted. Then, based on measured architectural parameters and joint properties, the relationship between sarcomere length and joint angle can be calculated. As an alternative to measurements in cadavers, sarcomere lengths can be measured in live humans using intraoperative laser diffraction [29] or less invasively with microendoscopy [30, 31]. Because sarcomere length strongly influences muscle force generation, an understanding of the relationship between sarcomere length change and movement has been used in many studies to provide added understanding of muscle design [29, 32–35].
The physiological cross-sectional area (PCSA) is the main architectural calculation. Theoretically, PCSA represents the sum of the cross-sectional areas of all the muscle fibers within the muscle, and thus it is the only architectural parameter that is directly proportional to the maximum tetanic tension generated by the muscle. The PCSA is almost never the same as the cross-sectional area of the muscle measured in any of the traditional anatomic planes, as would be obtained using a noninvasive imaging method such as MRI, CT, or ultrasonography. It is calculated as muscle volume divided by fiber length and has units of area. Because fibers may be oriented at a pennation angle relative to the axis of force generation, not all of the fiber tensile force is transmitted to the tendons. Specifically, if a muscle fiber is pulling with X units of force at a pennation angle θ relative to the muscle axis of force generation, only a component of muscle fiber force (X · cos θ) will actually be transmitted along the muscle axis. Thus, the volume/length is often multiplied by cosine θ (pennation angle) to yield PCSA. This is particularly important in the rotator cuff because muscle cross-sectional area is dominantly defined as an anatomical cross-sectional area at the level of the coracoid process on a coronal oblique MR image of the shoulder (Fig. 18.2). This is, of course, not a PCSA calculation and should not be extrapolated to an isometric force-producing capacity.


Fig. 18.2
T1-weighted sagittal oblique image of the rotator cuff muscles demonstrating mild fatty atrophy of the supraspinatus muscle
18.7.1 Anatomy and Architecture of the Rotator Cuff Muscles
As noted above, the fibers of the rotator cuff muscles are multipennate, meaning that they are organized obliquely to the internal tendon axis. This allows for more fibers to be packed into parallel, which yields larger force-generating capacities. The disparate organization of these fibers relative to the tendon axis has led some scientists to explore the possibility that each portion (or head) of these muscles has a unique function [36]. However, it is important to understand that muscle fibers converge on the internal muscle tendon prior to the formation of the more clearly defined, external tendon. Although it is possible that the muscle subregions influence forces on the tendon itself (e.g., the vasti in the patellofemoral joint), there is no evidence to suggest that there is slippage between regions of tendon, which could yield muscle subregion-specific actions at the joint. In fact, these studies artificially separate the tendon in order to demonstrate the unique behavior of a subregion.
Detailed characterization of the architectural features of these muscles has yielded some surprising results. First, all four rotator cuff muscles tended to have relative short muscle fibers, and many of them are expected to generate large isometric forces (Fig. 18.3) [37]. This indicates that the muscles are intended to generate relatively large forces over a relatively narrow range of lengths—essentially designed to stabilize the glenohumeral joint. Of course, their position on the scapulae allows them to maintain their length through a very wide range of joint positions. Second, as a component of the architectural measurements, muscle sarcomere lengths were measured in the anatomically neutral joint configuration. Interestingly, the supraspinatus and infraspinatus muscles had sarcomere lengths in the 3.2 μm range, which is significantly longer than the optimal sarcomere length in humans (2.7 μm) [37]. This indicates that supraspinatus and infraspinatus are generating passive tension (stabilizing the glenohumeral joint) even at rest! This passive tension is likely to be partially responsible for muscle retraction after tears. It also indicates that the muscles likely have abnormal mechanosensitivity because it is well known that muscles under chronic stretch increase fiber length through serial sarcomere addition, which these muscles do not seem to do [38, 39].


Fig. 18.3
Muscle fiber length (length changing capacity) and physiological cross-sectional area (isometric force-producing capacity) for the rotator cuff and deltoid muscles. The rotator cuff muscles have relatively short fibers compared to the deltoid and relatively large PCSAs, indicating that they are designed to produce large forces over a relatively narrow range of lengths
18.7.2 Architectural Adaptations to Tendon Injury
In the presence of rotator cuff tendon injury, atrophic muscle changes have been observed in both changes in animals and humans. Grossly, this can be seen in smaller anatomical cross-sectional areas and elevated intramuscular fat contents in the supraspinatus muscle (Fig. 18.4) [40]. Not surprisingly, this leads to impaired shoulder function and poor functional outcomes after repair [41]. Also, chronically torn muscles tend to be stiffer [42], which can lead to higher repair site tensions and failures [43], and these muscles tend to be resistant to hypertrophy even after successful tendon repair [44, 45]. Similarly to the radial loss of the muscle after tendon injury, muscle fiber lengths also decrease when muscles retract and do not appear to recover after repair [46]. This is critically important for post-repair muscle function, as short fibers, when repaired to the anatomical footprint, are not only likely to generate high repair site tension, but they are also going to change the position of the muscle on its length-tension curve. For example, pulling a muscle with a full-thickness tendon tear (and short muscle fibers) back to the anatomical footprint would likely pull its sarcomeres to very long lengths, which will impair the muscle’s ability to generate force (Fig. 18.5). Although we would expect these muscles to adapt by adding sarcomeres in series, this concept has never been tested in the rotator cuff.



Fig. 18.4
T1-weighted sagittal oblique image of the rotator cuff muscles demonstrating severe fatty atrophy of the supraspinatus and infraspinatus muscles

Fig. 18.5
Sarcomere length-tension curve demonstrating the approximate sarcomere operating range for each tear state (solid lines), using the operating ranges extrapolated in the case of FTT (Full-thickness tear) to account for diminished sarcomere number. MT (massive tear) is represented by a single point (red square), as this muscle is mechanically disconnected from the joint. The dotted lines demonstrate the theoretical, immediate post-repair sarcomere length operating ranges of FTT (blue) and MT (red) [46]
There is some debate about the connection between fatty atrophy and nerve injury in these muscles [47–49], which has been exacerbated by the widespread use of nerve injury to induce muscle atrophy in small animal models of rotator cuff disease [50–54]. At the present time, the best prospective data suggests that, although fatty atrophy is fairly common, suprascapular nerve injury is not even in large or massive tears [55]. However, the pattern of fatty infiltration is different in patients with neuropathy [56]. On the contrary, aging is associated with smaller muscle cross-sectional areas and higher fat content [57]. As such, the mechanisms behind the accumulation of fat in the muscle remain the focus of intense scientific work in this area.
As one might imagine, the accumulation of fat in these muscles poses significant challenges related to architectural definitions because these measurements typically rely on muscle mass and, therefore, do not discriminate between contractile and noncontractile materials.
18.8 Advanced Imaging of Muscle Structure
The presence of fatty atrophy and other atrophic changes in the muscle has given rise to quantitative volumetric imaging of the shoulder muscles [58]. These advanced MRI techniques are currently labor intensive, but they do provide a more comprehensive understanding of fat distribution and, therefore, volumetric muscle loss (Fig. 18.6). Similarly, advanced imaging techniques such as diffusion tensor MRI (DT-MRI) have the potential, when paired with tractography techniques, to yield estimates of raw muscle fiber length (Fig. 18.7). It is important, however, to understand that these estimates of muscle fiber length do not consider sarcomere lengths, which are shorter after tears [46]. This issue severely compromises the use of DT-MRI and will require further experimental work to resolve (Fig. 18.8).




Fig. 18.6
3D MRI reconstruction of a torn supraspinatus volume identifying the muscle (red) and fat (yellow). These data will, perhaps, yield more accurate quantitation of volumetric muscle loss after injury

Fig. 18.7
3D DT-MRI tractography reconstructions of a rotator cuff: supraspinatus (red), subscapularis (blue), and infraspinatus (green). These data will, perhaps, yield the first in vivo quantitation of muscle fiber length after injury and repair. Importantly, tractography images do not yield sarcomere length-corrected fiber length—a pitfall that will require further experimental work to resolve

Fig. 18.8
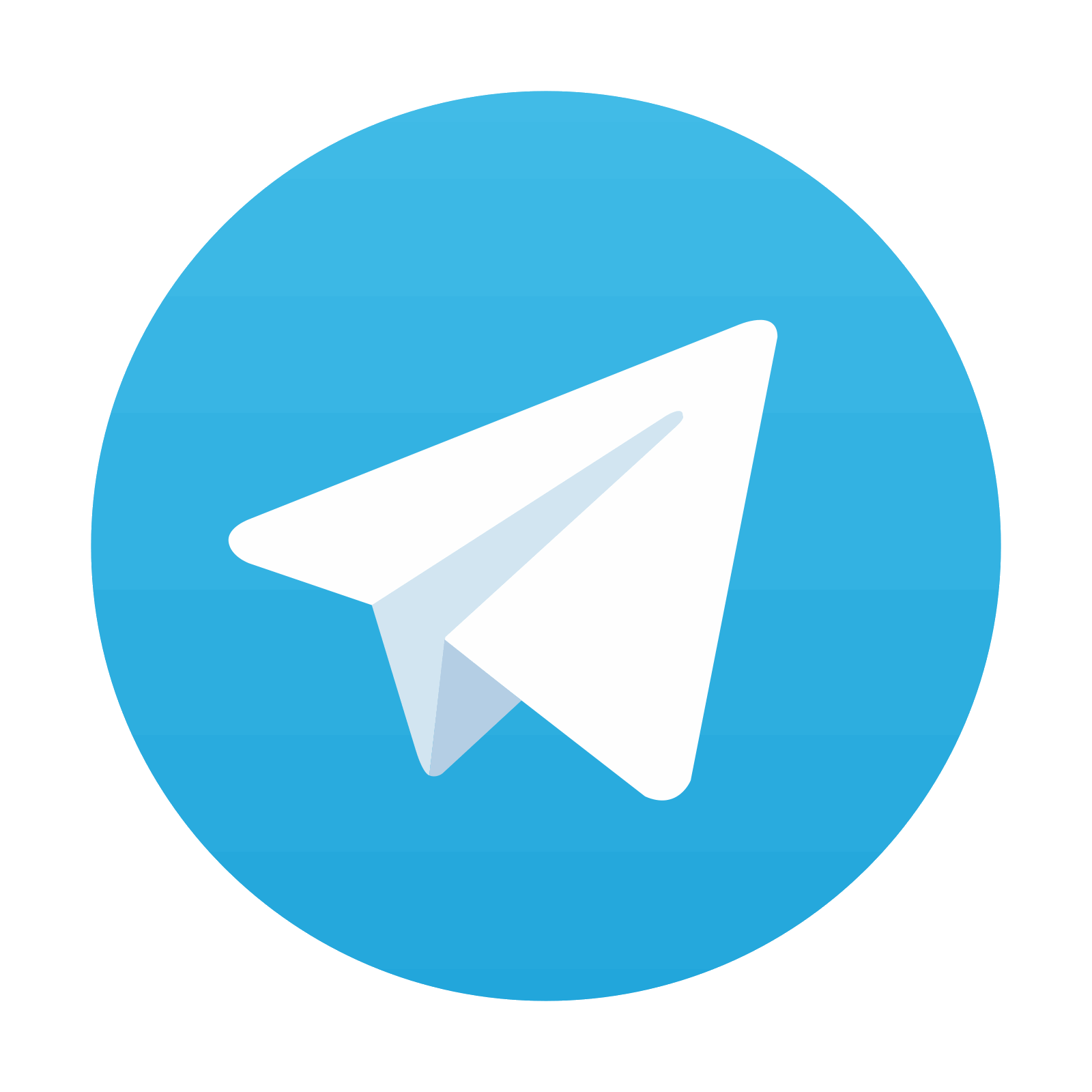
H&E stained rotator cuff muscle section demonstrating a single muscle fascicle (yellow border) with a central region (white arrowhead) in which adipose tissue has replaced degenerating muscle (white arrow) without altering the structure of the fascicle [59]
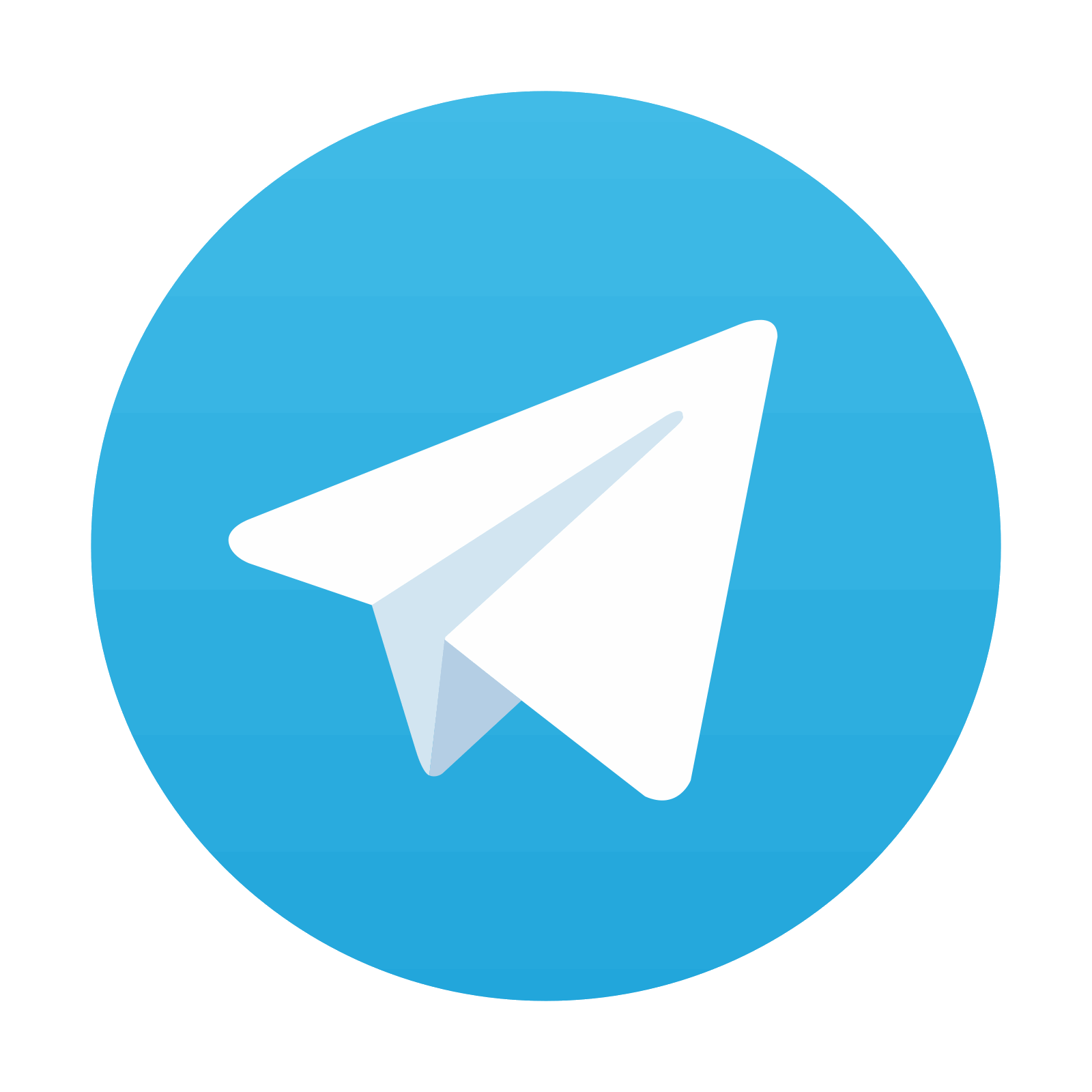
Stay updated, free articles. Join our Telegram channel
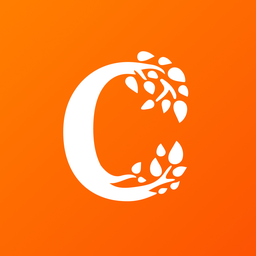
Full access? Get Clinical Tree
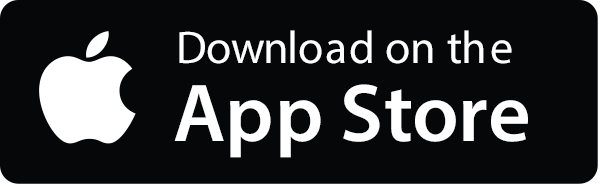
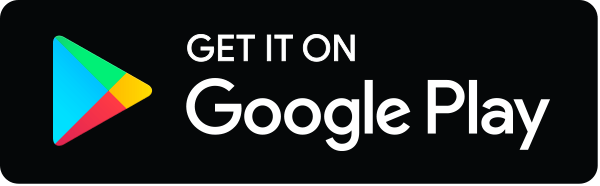
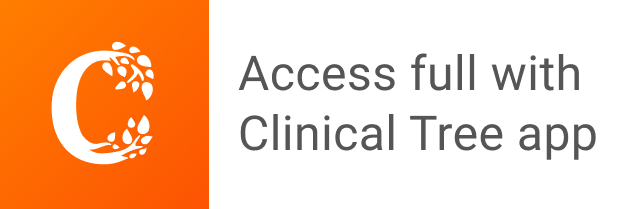