Fig. 43.1
Development of the basic TEC from human MSCs. (a) The monolayer sheet immediately after detachment from culture dish and (b) the actively contracted 3D tissue
43.4 Chondrogenic Differentiation Capacity of Human TECs
TECs derived from human MSC were cultured in a chondrogenic medium containing BMP-2 and showed increased glycosaminoglycan (GAG) synthesis and deposition as evidenced by intense Alcian blue staining (Fig. 43.2) [48]. Similarly, detection of cartilage-specific markers, collagen II (Col2a1), aggrecan, and sox9 messenger RNA (mRNA) by semiquantitative RT-PCR confirmed the cartilage-like phenotype of the TEC exposed to the differentiation medium.


Fig. 43.2
The histology of the TEC in control medium or in the chondrogenic medium stained with Alcian blue. Bar = 50 μm (upper). Bar = 300 μm (lower). Arrow showed that the nuclei were in lacuna. Quoted and modified from [48] (Ando et al., Tissue Eng Part A 2008)
43.5 The Adhesive Properties of Basic TEC
As mentioned above, due to its unique matrix organization, articular cartilage exhibits anti-adhesive properties, and therefore, integration of the implanted tissue to the adjacent cartilage normal matrix has been an issue in the treatment of chondral injuries [3]. To overcome this problem, most implantation procedures to repair chondral lesions require an enzyme treatment of the cartilage matrix surface [50], suture reinforcement of the initial fixation [51, 52], or by the use of absorbable pins [44]. However, an animal study revealed that a suture track in the surrounding articular cartilage remained unhealed, becoming a defect which could potentially be a trigger site for subsequent degradation of matrix around the margin, between the implant and the adjacent cartilage tissue [51]. Therefore, to avoid such potential complications, an implantable tissue that possesses highly adhesive properties to cartilage tissue is likely advantageous for securing tissue integration.
To test the adhesive property of TEC to a cartilage matrix, basic TECs were placed on the injured surfaces of porcine chondral defect. At 7 days after the implantation on the defects, the TECs were macroscopically adhered to the injured chondral surfaces without reinforcement of the fixation [49]. Histology of the postimplantation site showed that the TECs appeared to be tightly adhered to the injured chondral surfaces (Fig. 43.3a). Higher magnification revealed that the adhesion was mediated by matrix-to-matrix interaction, and fibronectin (Fig. 43.3b) and vitronectin (data not shown) were localized at the interface between the TEC and the surface of the chondral defects.


Fig. 43.3
The adhesiveness of basic TEC to cartilage matrix. The interface view of (a) H&E staining and (b) fibronectin staining of porcine chondral defect treated with TEC at day 7. Arrows indicate the interface between the TEC and the cartilage defect. Bar = 50 μm. Quoted and modified from [49] (Ando et al., Biomaterials 2007)
43.6 Cartilage Repair Using Basic Porcine TECs in Both Skeletally Mature and Immature Pigs
One of the crucial factors that may affect the results of cell-based therapies is the age of the donors and the recipients. Regarding the cell proliferation and differentiation capacities of MSCs, it is controversial as to whether they are age dependent [53–56] or not [16, 57–60]. In terms of host tissue reaction, natural healing responses of osteochondral defects have been compared between immature and mature rabbit models, demonstrating better healing responses in immature rabbits [61–64]. On the other hand, there has been lack of studies comparing the results of cell-based repair of chondral defects between immature and mature animal models. Regarding the use of a clinically relevant animal model for cartilage repair, it is difficult to create a chondral injury which does not breach the subchondral bone in small animals such as rabbits, rats, and mice due to the limited thickness of their articular cartilage, and therefore, these conditions may not be as clinically relevant to be used on larger animals. Thus, taking into consideration the clinical relevance, it is preferable to utilize a large animal model to investigate the influence of maturity on the results of cell-based therapies to repair chondral lesions. Therefore, in order to assess the efficacy of the TEC in an in vivo model, a porcine model was chosen as the physiology of the pig is similar, in many aspects, to humans [65]. Moreover, porcine articular cartilage of the knee is sufficiently thick to allow the creation of a chondral defect without damaging the subchondral bone. Prior to performing such studies, a preliminary characterization of the ability of porcine-derived MSC from synovium to generate a TEC comparable with the discussed above for the human MSC was undertaken.
To that end, we compared the in vitro characteristics of cell proliferation and chondrogenic capacity in porcine MSCs isolated from skeletally immature animals (3–4 months old) and mature animals (12 months old) and demonstrated that there were no significant differences in these capacities of porcine synovial MSCs derived from immature or mature animals [21].
To test the feasibility of using the porcine TEC approach for a wide range of recipient ages without chondrogenic manipulation to repair a chondral injury, immature and mature porcine chondral injury models were utilized in experimental studies. The TECs derived from the porcine synovial MSCs were implanted into equivalent chondral defects in the medial femoral condyle of both immature and mature pigs, respectively. At 6-month postimplantation, regardless of the starting age, untreated lesions exhibited no evidence for repair or partial tissue coverage, while the defects treated with a basic TEC were totally or primarily covered with repair tissue (Fig. 43.4a–d). The mean macroscopic scores for the TEC groups were significantly higher than those for the untreated groups, regardless of the maturity (Fig. 43.4e). In this situation, a lower score is suggestive of a failure to resolve the injury and progression toward overt osteoarthritis. Histologically, the chondral lesions in the nontreatment control groups showed evidence of osteoarthritic changes, with loss of cartilage and destruction of subchondral bone in both skeletally immature and mature animals (Fig. 43.5a, b). Conversely, when treated with a TEC, the defects were filled with repair tissue exhibiting good integration to the adjacent cartilage and the restoration of a smooth surface, regardless of age at the time of implantation (Fig. 43.5c, d). Notably, higher magnification views indicated that there was good tissue integration to the adjacent cartilage, obtained when the TECs were implanted in both immature and mature animals (Fig. 43.5f, g, arrows). The repair tissue exhibited predominantly spindle-shaped fibroblast-like cells in the superficial zone of the repair tissue, while the majority of the remaining repair matrix contained round-shaped cells in lacuna (Fig. 43.5e, h). Following implantation, no histological findings were obtained that suggested either central necrosis of the implanted TEC or an abnormal inflammatory macrophage and lymphocyte response consistent with some form of immunological rejection that had occurred in this allogeneic situation, regardless of the pigs’ age. Using histological scoring, the TEC groups exhibited significantly higher scores than the control group, regardless of the maturity (Fig. 43.5i). Comparing the repair tissue development following TEC implantation in immature and mature animals, no significant differences were detected.



Fig. 43.4
Macroscopic view of immature (a, b) or mature (c, d) porcine chondral lesion treated without (a, c) or with the TEC (b, d) at 6 months post operation. Bar = 10 mm. (e) Macroscopic score of the chondral lesion treated with the TEC or untreated at 6 months. Regardless of skeletal maturity, the TEC group shows significantly higher score than the untreated group. * p < 0.05. Quoted and modified from [21] (Shimomura et al., Biomaterials 2010)

Fig. 43.5
Safranin O staining of untreated chondral lesions (a, b) or lesions repaired by the TEC (c, d). (a, c) immature recipients. (b, d) mature recipients. Bar = 1 mm. Higher magnification view at the interface (f, g) and center (e, h) area of the repaired tissue by the TEC. Bar = 200 μm. Regardless of the maturity, the defects treated with the TEC are completely filled with Safranin O-positive repaired tissue (c, d) with good tissue integration (f, g, arrow). (i) Modified ICRS score in repaired cartilage immature and mature recipients. The TEC group exhibits significantly higher scores than does the untreated control group, regardless of the maturity. *p < 0.05, **p < 0.01. Quoted and modified from [21] (Shimomura et al., Biomaterials 2010)
In addition, the mechanical properties of porcine chondral defects treated with a porcine-derived TEC were assessed at 6-month postimplantation in immature and mature animals, in comparison with those of normal cartilage. Regarding the mechanical properties of articular cartilage, it should be considered that articular cartilage is a biphasic viscoelastic material that exhibits strain-rate dependent mechanical behavior [66]. It means that the viscoelasticity of cartilage which retains interstitial water might be mainly reflected in faster compression test, while the matrix viscoelasticity without interstitial water could be mainly reflected in slower compression tests. Within the tissue localized in the defects of the untreated control group, the tangent modulus (defined as the slope of the curve at 5% strain) in immature animals was significantly lower than normal cartilage at a compression rate of either 4 μm/s (Fig. 43.6a) or 100 μm/s (Fig. 43.6b). In contrast, there were no significant differences detected between the tangent modulus for the repair tissue resulting from implantation of a TEC and normal cartilage at either 4 μm/s (Fig. 43.6a) or 100 μm/s (Fig. 43.6b) in immature animals. Similarly, the mean tangent modulus in the untreated mature animals was significantly lower than normal cartilage at a compression rate of 4 μm/s (Fig. 43.6a), while there were no significant differences detected between the tangent modulus for repair tissue in mature recipients treated with a TEC and normal cartilage at either 4 μm/s (Fig. 43.6a) or 100 μm/s (Fig. 43.6b). These results suggest that the viscoelastic properties of the defects’ repaired tissue by TEC implantation are likely similar to those of normal cartilage, regardless of age at the time of implantation.


Fig. 43.6
The results of compression tests at slower compression speed (4 μm/s) (a) and at faster compression speed (100 μm/s) (b). Regardless of skeletal maturity, there is no significant difference detected in the tangent modulus of the repaired tissue by the TEC and of normal cartilage at either slower or faster compression speed. Conversely, untreated cartilage, whether immature or mature, showed significantly lower tangent modulus than normal cartilage at either slower or faster compression speed. * p < 0.05. Quoted and modified from [21] (Shimomura et al., Biomaterials 2010)
43.7 Clinical Trials Using a TEC Derived from Human Synovial MSCs for Repairing Isolated Cartilage Defects
Based on the encouraging results of the preclinical studies discussed above, we have proceeded to clinical studies under the auspices of an approved first in man protocol [67]. Patients with symptomatic chondral lesions of the knee and who meet the inclusion criteria (isolated chondral lesion ≦ 5 cm2, 20–60 years of age, with normal alignment) were enrolled (Fig. 43.7a). Under general or spinal anesthesia, approximately 1 g of synovial membrane is harvested from the knee joint, which is then subjected to the isolation and culture of MSC for their separation and expansion. Following 3–5 weeks post-tissue harvest, the TECs are prepared for autologous implantation. By mini-arthrotomy or arthroscopy, the chondral lesion is debrided to prevent breaching the subchondral bone. Before implantation, the TEC is washed several times with sterile phosphate-buffered saline to minimize calf serum–related protein contamination, followed by the adjustment of the TEC size to match that of the chondral defect. Implantation is completed within 5–10 min, without any reinforcement for fixation. The knee is immobilized with a brace for 2 weeks followed by the initiation of range-of-motion and muscle strengthening exercises. Full weight bearing is allowed at 6–8 weeks after implantation surgery. Return to strenuous activity is allowed approximately 12 months following the implantation. The duration for follow-up is 1 year, and the primary end point of this study is an analysis of adverse reactions. The secondary end point is the assessment of feasibility, which consists of subjective assessment (visual analog score [VAS] for pain and knee injury and osteoarthritis outcome score [KOOS]), and structural assessment. For the structural assessment, histological analysis of a biopsy specimen at 12 months and magnetic resonance imaging (MRI, conventional and quantitative such as T2 mapping) at 3, 6, and 12 months are performed. The preliminary results indicated that this treatment restored normal joint function by completely covering the cartilage defect with cartilage-like repair tissue with second-look arthroscopy (Fig. 43.7b) as well as high T2 mapping profile (Fig. 43.7c, d) 12 months after the implantation without any serious adverse events. Also, the biopsy specimen obtained from implanted site exhibited the well-organized hyaline cartilage-like tissue with positive Safranin O staining (Fig. 43.7e). Similar to the results of the structural assessments, the subjective assessments by VAS and KOOS were improved.


Fig. 43.7
Arthroscopic (a, b) and MRI (c, d) analyses of repair tissue following implantation of a tissue-engineered construct (TEC) to repair human chondral defects in clinical trial. Arthroscopic views of the pre-operation defect (a) and then 12 months after implantation of a TEC (b). The cartilage defect was completely covered with a cartilage-like repair tissue. T2-weighted mapping of the lesion at the femoral groove before implantation (c) and 12 months after implantation (d). (e) Biopsy specimen 12 months after the implantation. Safranin O staining reveals a well-organized hyaline cartilage-like tissue. Quoted and modified from [47] (Shimomura et al., Cartilage 2015)
43.8 TEC Derived from Synovial MSC to Regenerate Cartilage and Its Potential Application to Other Musculoskeletal Tissues
The present chapter has demonstrated the feasibility of using a unique scaffold-free TEC generated from synovial MSCs for effective cell-based cartilage repair. The cultured synovium-derived cells had high self-renewal capacity and stable expression profiles for surface antigen characteristics through passage 4–7, similar to what has been observed for bone marrow-derived MSCs. Furthermore, these cells retained the capability for osteogenic, chondrogenic, and adipogenic differentiation as previously reported [16]. Thus, the cultured synovium-derived cells display characteristics similar to those of MSCs derived from other sources, and, consequently, the in vitro generated TEC could be regarded as an MSC-based 3D bioengineered tissue.
The development of a 3D tissue without an artificial scaffold is the crucial center of this tissue engineering technology, a technology that is based on the active contraction of a cultured monolayer cell/matrix complex. Importantly, the TEC develops without any exogenous scaffold, and therefore, implantation of the TEC would have minimal risk of potential side effects induced by artificial or extrinsic biological materials contained in a scaffold. Furthermore, we confirmed that human serum is no less effective than bovine serum in promoting proliferation of synovium-derived MSCs without losing the differentiation potential of the cells [46]. Accordingly, with the use of autologous human serum, it is technically possible to develop the TEC in a xeno-free system. A set of circumstances which would minimize the risk of infectious agents, as well as the immune reactivity, developing to the TEC via of any associated xeno-proteins [39].
Another further structural advantage of the TEC is that the MSCs and the ECM synthesized by the cells are integrated together into a 3D structure with a uniform cellular distribution. Thus, there is no need to modify or adjust the cellular distribution within the TEC. It is also notable that the TEC possesses sufficiently self-supporting mechanical properties although it does not contain an artificial scaffold. The tensile strength of the TEC became 1.32 ± 0.25 MPa after the 21 days culture in the presence of Asc-2P [48], which is comparable with healing of the ligament tissue at 1–2 weeks after injury [68]. Therefore, such TEC can be readily handled without causing overt damage to the matrix during implantation procedures. Moreover, such TEC with high tensile strength may be applicable to tendon and/or ligament repair, which will be exposed to mechanical stress during the repair process.
Another important biological characteristic of the TEC described in this report is its tissue adhesiveness. This property contributes to the rapid and secure adhesion of the TEC to a natural cartilage matrix, and thus, simple implantation procedures for the placement of the TEC into chondral lesions or defects could be expected to proceed without augmentation of the initial fixation. Moreover, such adhesiveness also enables rapid self-association internally with its own matrix, a factor which likely contributes to the tissue plasticity of the TEC. In reality, it is thus possible to develop a spherical-shaped tissue several millimeters thick by allowing the released monolayers from several dishes to fold in series. With such “plasticity,” it is possible to develop a TEC that matches the needed size and shape to repair a chondral defect more than several millimeters in thickness. Although we have not yet identified the crucial factor(s) which determine the TEC tissue adhesiveness, immunohistochemical analysis has shown that fibronectin and vitronectin are localized at the interface between the TEC and the base of the chondral lesions. Therefore, fibronectin and vitronectin may likely be, at least partially, involved in the adhesive properties of these in vitro generated TECs.
It is known that a 3D culture environment at high density, such as in micromass cultures [69] or pellet cultures [70], is an important variable to promote chondrogenesis. However, these methods cannot be directly applied to most clinical situations due to limitations related to the material’s mass size [25]. The present studies revealed that the TEC approach overcomes this problem of tissue size while providing a 3D and highly dense environment for the MSCs to differentiate toward a chondrogenic phenotype following implantation without leading to cell and tissue necrosis. The TEC originally does not contain chondrogenic marker molecules such as collagen II and instead is rich in collagen I and III. However, following implantation in vivo, the basic TEC which did not receive ex vivo stimulation toward chondrogenic differentiation appears to have responded to the endogenous environment and evolved a matrix composition similar to chondrogenic tissue. The findings from the in vitro chondrogenesis experiments suggest that local biological and mechanical environment factors may facilitate the degradation of the “old” matrix followed by the synthesis of a new chondrogenic matrix. Thus, during the postimplantation chondrogenic differentiation in vivo, it leads to an overall phenotypic change of the matrix within the TEC.
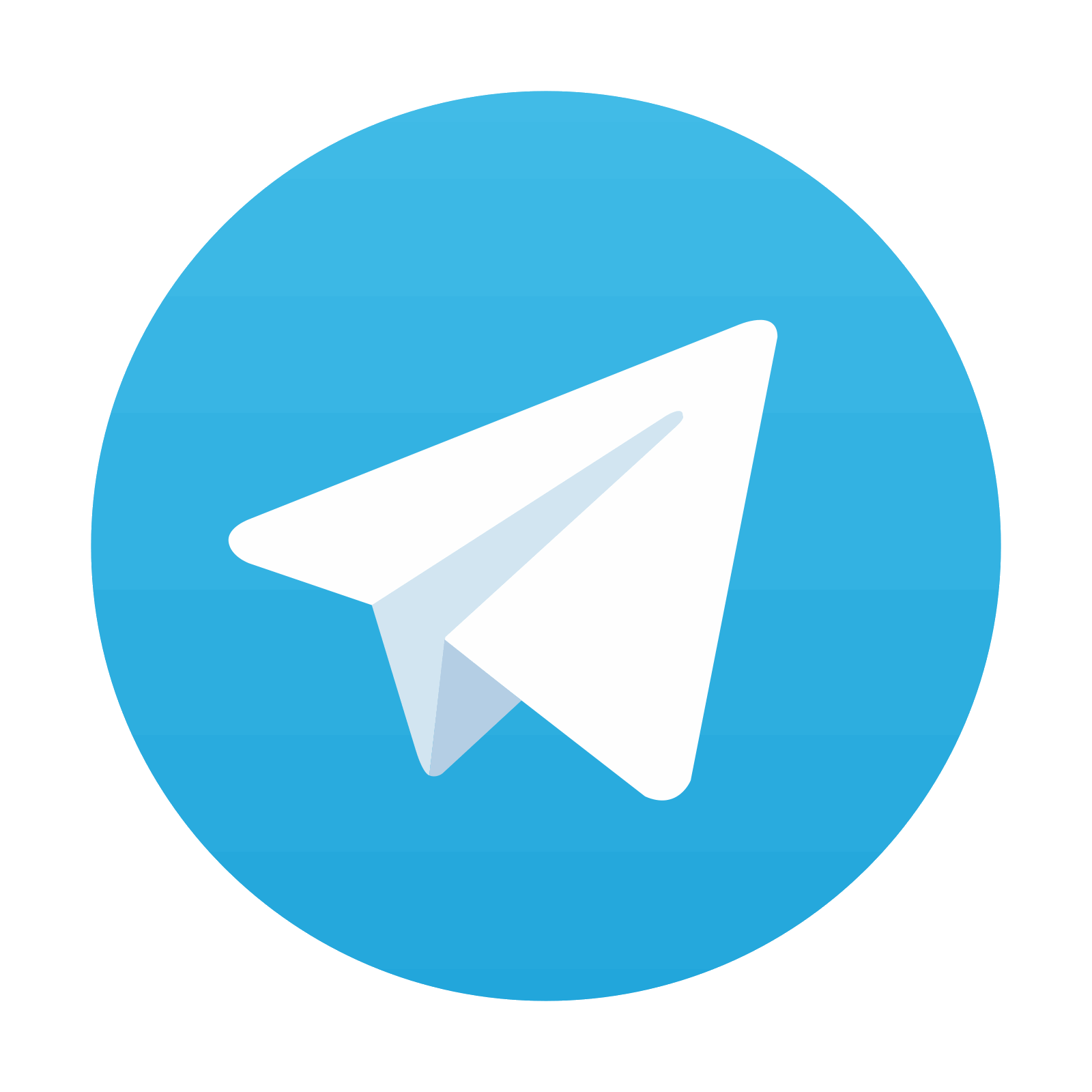
Stay updated, free articles. Join our Telegram channel
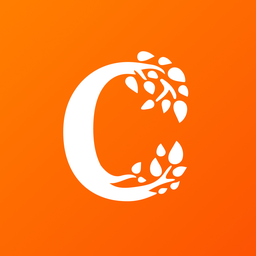
Full access? Get Clinical Tree
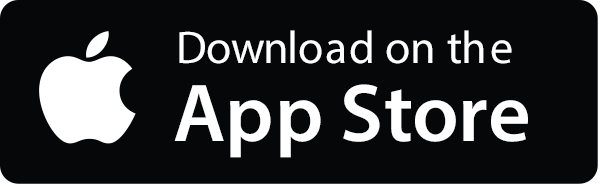
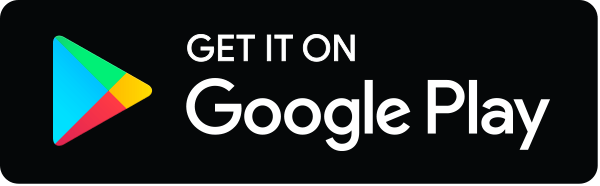