CHAPTER 67 Principles of Bone Fusion
Spinal fusion may be defined as a bony union between two vertebral bodies following surgical manipulation. Spinal fusion was first reported in 1911 for treatment of Pott disease. The mechanical stability provided by fusion was intended to inhibit progressive deformity and the spread of the tuberculous infection.1 Surgery to accomplish spinal fusion has now been extended to treat a variety of spinal conditions including scoliosis, kyphosis, fracture, dislocation, spondylolisthesis, and intervertebral disc disease.
Much has changed since the pioneering efforts of Albee1 and Hibbs2 in the early part of this century. Specialized techniques and surgical approaches have been developed for internal fixation and fusion of every part of the spine. Additionally, there have been significant advances in diagnostic techniques, intraoperative image guidance, intraoperative monitoring, minimally invasive surgical approaches, and bone graft materials. These advances have allowed for the aggressive correction of many severe spinal deformities with relative safety and predictability. Furthermore, the biologic principles on which these procedures are based have become increasingly better understood and used.
All fusion surgery involves preparation of bony surfaces at the site of the intended fusion. This usually involves the removal of soft tissues and decortication of bony surfaces. The stimulus for the bone healing response, commonly referred to as the “bone graft,” may be autologous or homologous bone (i.e., allograft bone) or one of an increasing number of synthetic materials or bioactive substances. As the graft is incorporated, bone tissue is formed by osteogenic cells. Union is accomplished when the newly synthesized bone matrix becomes mineralized and remodels with mature bone, having sufficient strength to bear physiologic loads without injury, thus becoming mechanically contiguous with the local host bone. Failure of bone formation, union, or effective remodeling results in pseudoarthrosis. The incidence of pseudoarthrosis ranges from 5% to 34% in large adult series.3–9
Fusions Site
Bone growth between vertebrae in a spinal fusion, as in all bone healing, is a cellular process, and unless cells are added to the fusion site, the tissues at the site are the only source of viable cells. Conventional autogenous bone grafts add osteogenic cells; however, it has long been recognized that only a small fraction of these cells survive.10–12 Consequently, preparation of the fusion site and handling of the tissue bed are of paramount importance for a successful arthrodesis. The components of the tissue bed that contribute most to the healing process are the local population of osteogenic stem cells and progenitor cells, local vascular tissues, the cells contributing to the inflammatory response, and the formation of a stable clot within the void spaces of the graft site. Bone, fat, and muscle have all been shown to contain osteogenic stem cells and progenitors that can contribute to new bone formation.13–20 These basic elements of the graft bed may be affected by local or systemic disease. However, the quality of these elements within the graft site is largely determined by surgical technique.
To preserve the local blood supply, the surgeon must attempt to minimize trauma to the host tissue bed imposed by the trauma of retraction, cautery, or desiccation. Any avascular, nonviable, or heavily traumatized tissues should be removed. The importance of the local blood supply cannot be overstated. The blood supply serves as (1) a source of oxygen and other nutrients to the healing tissue, as well as control of local pH; (2) a vehicle for endocrine stimulation; (3) a conduit for recruitment of inflammatory cells, which both produce paracrine factors, which may mediate the early proliferation of osteoblastic progenitors cells and serve to reduce the potential for infection; (4) a source of endothelial cells that produce paracrine factors, which may enhance osteoblastic differentiation21; and (5) a potential source of osteoblastic progenitors in the form of the vascular pericyte22 or circulating osteogenic cells.23
The effect of the postoperative hematoma on the success of fusion has been debated. It has been suggested that spinal fusion wounds should not be drained because the fibrin-rich local hematoma may provide an osteoconductive scaffold or matrix, which may assist some of the initial phases of bone healing. Additionally, the trapped platelets in the hematoma release platelet-derived growth factor (PDGF), epidermal growth factor (EGF), basic fibroblast growth factor (bFGF or FGF-2), vascular endothelial growth factors (VEGF), transforming growth factor beta (TGF-β), and other growth factors that play a critical role during the repair process (see later).24 On the other hand, the presence of a large hematoma may displace some of the vascular tissue surrounding the graft site away from the graft, slowing the vascularization of the graft site. It may also increase the chance for displacement of the graft and the potential for nonunion or bone formation outside the intended site.
The inflammatory response in the wound site and the grafted bed represents a critical event in the healing process. This response will involve the removal of necrotic tissue debris, lysis of the local fibrin clot, the establishment and reestablishment of a vascular supply to the graft and host tissue, and synthesis of an early matrix rich in hyaluronic acid.12,25–28 After the surgical procedure, polymorphonuclear cells, lymphocytes, monocytes, and macrophages migrate to the fusion site and perform their various functions. Among these, and possibly the most important in terms of affecting vascular endothelial cells and osteoblastic progenitors in the graft site, is the local production of paracrine signals: cytokines, kinins, and prostaglandins. These messages act as chemotactic signals and growth factors, affecting the proliferation, migration, differentiation, and activity of a variety of cells, as well as modulation of local blood flow, vascular permeability, and angiogenic response of local endothelial cells. In this way, the inflammatory response establishes the local environment in which the early events of the bone healing response occur. It is not surprising, therefore, that agents that inhibit the inflammatory response have been shown to alter or inhibit bone healing.29–34
In addition to the influence of the local blood supply and the inflammatory response, the host bone surface itself is known to have a profound effect on the healing process in spinal fusions. When properly prepared by the surgeon, local bone will serve as a reservoir of osteogenic cells and osteoinductive signals. It also provides an osteoconductive surface for graft incorporation and serves as part of the local blood supply to the graft site. As such, the goal of surgical preparation of local bone is to minimize cellular and mechanical damage to the host bone, while maximizing the availability of osteoprogenitor cells and the osteoconductive and osteoinductive properties of this surface. This is generally achieved by subperiosteal dissection with or without decortication or roughening of the underlying bone to expose vascular osteonal or endosteal bone spaces. Decortication can be achieved with manual tools such as a rongeur or osteotome. Alternatively, a power bur may be used, provided caution is exercised to prevent thermal necrosis of the bone owing to the heat of friction at the site by using continuous irrigation and limiting periods of contact between the bur and bone at any one site.35
The surface area of cancellous bone exposed during decortication is another factor thought to affect the success of a spinal fusion. Increasing the available surface area also increases the number of osteogenic cells at the fusion site, which should have a positive effect on the amount of bone formed and the rate of graft incorporation. Additionally, an increase in the osteoconductive surface area available should increase in the area of contact between the osteogenic host bone and the graft material and potentially lead to greater mechanical strength of the subsequent bony union. This may account for the greater success of allografts in anterior fusions36 as compared with posterior fusions, which generally rely on a smaller area of decorticated bone per fusion segment. Similarly, this may also contribute to the lower fusion rates seen in myelomeningocele,37,38 where the laminae are not available as a surface for fusion.
In theory, any exposure of the local bone surface, osteonal spaces, or marrow spaces that does not excessively weaken the mechanical strength of local bone should increase the number of osteoblastic progenitors with access to the graft site.39
Bone Graft
All bone grafting strategies involve either the transplantation or targeting of osteoblastic stem cells or progenitor cells. Bone grafts have been described as having osteogenic, osteoinductive, and osteoconductive properties. Grafts may also contribute to mechanical stability and vascularity at the graft site.40 Detailed reviews of the principles underlying these practical clinical concepts have been recently published.41–43
In contrast to osteoinduction, osteoconduction is the result of the structural and surface features of a graft matrix. Osteoconductivity refers to the capacity of a graft matrix to enhance the attachment, migration, proliferation, and differentiation of osteoblastic stem cells and progenitors, as well as other cells that contribute to the bone healing response. As a result, osteoconduction promotes the distribution of a bone healing response of the graft. The osteoconductivity of a matrix is a function of its macrostructure or architecture, the size and connection between pores on the material, and its surface chemistry and surface texture. In the case of degradable materials, the degradation properties of the material are also critical, specifically the degradation rate, the chemical species that are released by degradation, and their rate of clearance from the site.41
Autologous Cancellous Bone
Autologous cancellous bone has traditionally been considered to be the “gold standard” of graft materials. Autograft has maintained a track record as the most reliable and effective graft material, particularly in the challenging clinical setting of spinal fusion.44–47 This finding is reinforced by the recognition that an autogenous cancellous graft provides all three areas of functionality: osteogenic bone and marrow cells; an osteoconductive matrix of collagen, mineral, and matrix proteins; and a spectrum of osteoinductive proteins provided within the transplanted matrix of cells.
The principle disadvantage of autogenous cancellous bone graft relates to the process of graft harvest. Autograft harvest adds operative time, pain, and blood loss, and it carries an increased risk of infection, cutaneous nerve damage, and even local fracture. Autograft harvest leaves the patient with permanent scars and a risk of long-term pain at the graft site. Increased blood loss attributable to the graft harvest results in an increased potential exposure to blood products along with all the associated costs and risks of transfusion. The incidence of major complications associated with the harvest of iliac crest bone graft has been reported to be 5% to 10%.48–50 The time, effort, and complications associated with autograft harvest are also associated with tangible costs to the health care system, which have been estimated in the range of $700 to $2200, not including the pain and scars. A second disadvantage of autograft is that, in addition to its cost and morbidity, the amount of autogenous bone is limited and may be insufficient in many settings, particularly in children undergoing arthrodesis over multiple segments.
Finally, autogenous bone has a biologic limitation as a cellular graft. Although cancellous bone from the pelvis is the most abundant source of osteoblastic stem cells and progenitors, these cells represent only about 1 in 20,000 cells in normal bone marrow, which is packed with many other cells that do not necessarily contribute to bone healing. When autogenous cancellous bone is harvested, its diverse mixture of highly metabolic cells is dissociated from its blood supply and is then implanted under conditions where it must compete with all of the other cells in bone and marrow for the limited amount of oxygen and other nutrients that are available to diffuse into the graft site. The metabolic demand within the graft site far exceeds the capacity for nutrient diffusion. This results in profound hypoxia as one moves more deeply into the graft. As a result, only cells within 1 to 2 mm of the surface of an autograft are able to survive transplantation. This fact was recognized by Burwell from histologic assessment in the 1960s and can now be defined in more quantitative terms on the basis of chemical engineering principles.41 Necrosis within the graft then places an additional burden on the site. Necrotic debris must be removed before new bone formation can occur. In addition, cell debris and the cytokines that are released by dying cells escalate the local inflammatory response, bringing in additional cells that further increase local metabolic demand.
Autologous Cortical Bone
The only advantage of cortical bone versus cancellous bone and other graft materials is its superior mechanical strength and the availability of cortical segments of sufficient size to fill virtually any skeletal defect. The ability to provide immediate mechanical strength at the time of implantation is a critical advantage in many situations, particularly in anterior interbody fusions. However, the mechanical strength of a cortical graft is not constant over time. Allograft bone is remodeled by the process of creeping substitution, resulting in increased porosity and progressive loss of strength during the first 12 to 24 months after implantation before remodeling and new bone formation reconstitute the mechanical properties of the grafted segment.40,51,52 This is associated with increased risk of graft failure and collapse during the first 24 months after implantation.
Combined grafts consisting of intact cortical and cancellous bone from the iliac crest are common and readily available graft materials with good mechanical properties and biologic properties of incorporation. The mechanical strength of these grafts is variable, however. Grafts from the anterior crest exhibit greater mechanical compressive strength than grafts from the posterior crest.53
Vascularized Autologous Grafts
Vascularized grafts are used extensively in many centers for musculoskeletal reconstructive procedures. High rates of vascular patency can be achieved by experienced microsurgeons. Many studies have shown clear advantages to using vascularized grafts in a number of settings.54–57 In anterior spinal fusions, donor vessels are available to support the vascularized graft. Suitable grafts with good mechanical strength are available from the anterior iliac crest, posterior iliac crest,58 fibula,59 or rib.60 In addition, an iliac graft pedicle flap on quadratus lumborum has also been described.61 In intrathoracic procedures, a vascularized rib graft may be mobilized on its intercostal pedicle, with limited additional morbidity and in much less time than a free vascularized graft.62,63 However, a rib graft provides less mechanical strength when compared with the iliac crest or fibula64 and therefore must be mechanically supplemented by additional cortical bone or internal fixation.
Autologous Bone Marrow
Bone marrow is a valuable and easily accessible source of osteogenic cells that is probably underused in contemporary clinical practice. The osteogenic potential of transplanted bone marrow was first documented by Goujon in 1869,65 and later by Senn in 1889.66 Studies by Burwell in the 1960s concluded that the formation of new bone following autografting resulted from the differentiation of osteogenic precursor cells contained within the marrow, in addition to osteoblasts on the surface of the graft material itself.67 Burwell postulated that following transplantation, these reticular cells free themselves from the sinusoidal walls to become primitive migratory cells; they then differentiate into osteogenic cells when they are exposed to osteoinductive substances released from the necrotic portion of the graft,68 or perhaps from osteoinductive materials contained within or secreted by the marrow itself.
Many studies have demonstrated the ability of marrow cells to form bone intramuscularly,69,70 subcutaneously,71 interperitoneally,72–74 in the anterior chamber of eye,75 and orthotopically. Using a suspension of marrow cells in diffusion chambers, Friedenstein showed that hematopoietic cells die following transplantation, whereas fibroblasts and other stromal elements are more resistant to hypoxia and may proliferate close to the surface of the graft to produce immature bone, suggesting the presence of an undifferentiated precursor cell in postnatal marrow.71,74,76,77 It has been well established that the osteogenic cells that contribute to this response are not derived from one homogeneous population of cells, but rather appear to come from two or more compartments of stem cells or progenitor cells that are upstream from the osteoblastic population in bone.43,71,78,79 A more mature preosteoblastic cell appears to be localizable to the marrow space adjacent to trabecular or osteonal bone. One or more populations of less mature and potentially multipotent cells appear to be present in the more liquid phase of bone marrow, possibly including cells associated with perivascular tissue of vascular sinusoids or other marrow vessels.
Osteoblastic differentiation proceeds in a series of steps, which can be conceptually divided into phases.80,81 An initial proliferative phase is characterized by expression of H4 histone, c-fos, and c-jun. A matrix synthesis phase is characterized by a reduction in proliferation and upregulation of gene products for type I collagen, osteopontin, osteonectin, and alkaline phosphatase. Finally, a matrix mineralization phase culminates in an osteoblastic phenotype characterized by expression of osteocalcin, bone sialoprotein, and responsiveness to 1,25-dihydroxyvitamin D and parathyroid hormone. A conceptual summary of the large body of literature related to osteoblastic differentiation is presented in Figure 67–1.43,82
The value of bone marrow as a bone graft, used alone or as a component in a composite bone graft material, has been supported by numerous studies in rats and rabbits.68,71,74–76,83–90 Lane and colleagues91 demonstrated the efficacy of autogenous bone marrow grafting in a 5-mm rat femoral defect and showed that the efficacy of bone marrow grafts were dependent on transplantation of viable cells. Yasko and colleagues92 also showed that bone marrow enhanced the performance of an effective synthetic BMP-2 material in rats, a finding consistent with prior reports on less pure BMP materials.88 However, in contrast to clinical practice, almost all of these studies in rodents have used bone marrow obtained by open harvesting of bone and/or irrigation of bone explants, rather than by aspiration.
Increasing evaluation of bone marrow grafting has been carried out in larger nonrodent models. Johnson and colleagues93 found canine bone marrow much less osteogenic than rabbit marrow when transplanted in diffusion chambers. Using a canine tibial model, Tiedeman and colleagues89 found that the percutaneous injection of marrow mixed with demineralized bone matrix powder produced overall results comparable with open cancellous grafting.
A few uncontrolled clinical series also imply that aspirated bone marrow can improve bone healing.68,94–97 Connolly reported successful treatment of 18 of 20 nonunions treated with casting or intramedullary nails plus percutaneous marrow injection. Healy and colleagues85 reported healing in five of eight delayed or nonunions of allograft host junction sites using marrow injection alone.
Recognizing the potential biologic values, many surgeons currently use bone marrow as an adjuvant to allograft bone grafts. This practice is currently supported primarily because the risk and morbidity of bone marrow aspiration from the iliac crest is low. The prospective trials needed to document the value or limitations of bone marrow grafting are only now being organized. However, these are informed by a significant volume of clinical information regarding methods and cellular yield of bone marrow aspiration98–100 and by a robust set of preclinical studies.101–105
The method of bone marrow aspiration has a significant effect on the concentration and prevalence of bone marrow–derived osteogenic cells. Muschler and colleagues,106 in a cohort of normal subjects undergoing elective orthopaedic procedures, showed that a mean of approximately 2100 osteoblastic progenitors (colony-forming units or CFU-Os) could be harvested in a 2-mL aspirate of human bone marrow from the iliac crest and that the mean prevalence of CFU-Os among nucleated marrow cells was approximately 1 in 37,000 cells. They further documented that the yield of CFU-Os harvested dropped rapidly as the volume of bone marrow aspirated was increased, owing to dilution with peripheral blood. On the basis of these findings, they recommended that aspiration of marrow be limited to 2 mL from each aspiration site in order to maximize the concentration of CFU-Os in the marrow graft. Further studies have demonstrated that the yield of osteoblastic stem cells and progenitors tends to decrease with age and that the prevalence of these cells may decrease more rapidly in women than in men.98,100,107–109 However, these data also show that there is marked variation from individual to individual in the cellularity of marrow and the prevalence of osteogenic cells that is not associated with age or gender.
McLain and colleagues110 obtained transpedicular aspirates from the vertebral bodies of 21 adults undergoing posterior lumbar arthrodesis and pedicle screw instrumentation. Aspirates were obtained from two depths within the vertebral body and were quantified relative to matched, bilateral aspirates from the iliac crest that were obtained from the same patient at the same time and served as a control. Aspirates of vertebral marrow demonstrated comparable or greater concentrations of progenitor cells compared with matched controls from the iliac crest. Progenitor cell concentrations were consistently higher than matched controls from the iliac crest (P = 0.05). The concentration of osteogenic progenitor cells was, on average, 71% higher in the vertebral aspirates than in the paired iliac crest samples (P = 0.05). With the numbers available, there were no significant differences relative to vertebral body level, the side aspirated, the depth of aspiration, or gender. An age-related decline in cellularity was suggested for the iliac crest aspirates. The authors concluded that the vertebral body is a suitable site for aspiration of bone marrow for graft augmentation during spinal arthrodesis.
One clinical grafting product, a collagen ceramic composite called Healos, has been released specifically for use as a delivery system for bone marrow–derived cells and has demonstrated strong clinical performance in terms of spinal fusion rates for both interbody and posterolateral fusion.111,112
Several authors have addressed the potential value of harvesting bone marrow by aspiration and then processing the cells that are collected to concentrate those that are most likely to be of value, thus eliminating those that are not. Connolly and colleagues83 described concentration of marrow-derived cells using centrifugation techniques and showed that a threefold to fourfold increase in the concentration of nucleated cells increased the amount of bone formation in a diffusion chamber in the rabbit. More recently, methods have been described that allow rapid intraoperative concentration and selection of osteoblastic stem cells and progenitors from bone marrow using an appropriately designed implantable allograft matrix as an affinity column to select osteoblastic cells on the basis of attachment behavior.113 This strategy has demonstrated increased bone formation, union rate, and mechanical performance in a validated canine posterior spinal fusion model. Performance of these concentrated grafts of marrow-derived cells appears comparable with that of autogenous cancellous bone, when cells are transplanted in an environment that includes a blood or marrow clot. The same strategy has been reported by Kadiyala and colleagues102,103 demonstrating union of a 5-cm canine femoral defect with both a rate and an outcome equal to autogenous cancellous bone. An early clinical cohort study applying bone marrow concentration strategies to lumbar interbody fusion has also reported a fusion rate of 85%, comparable with historical autograft controls.114
Muschler and colleagues41–43 have recently published three more detailed reviews of the biologic principles and practical strategies for harvest and use of stem cells and progenitor cells for bone healing applications. Other strategies such as the use of these cells as the delivery system or as the target cells for gene therapy applications are addressed in other chapters in this text.115–122
Structural Allografts and Cages
Use of allograft bone has been well characterized over the past 30 years.123 There are four principal advantages of allografts. First, they eliminate the morbidity associated with harvesting autologous bone. Second, and in contrast to autograft bone, the volume of available allograft is essentially unlimited. Third, because cortical allografts can be selected from any bone (not just the iliac crest or tibial hemicortex), they provide the surgeon with access to grafts that have mechanical strength and options for shaping that are superior to any autograft site. Fourth, allograft bone can be preprocessed into a wide range of specialized physical forms (e.g., blocks, threaded or nonthreaded dowels, sized rings and wedges, chips, fibers, powder) prepared from cortical and/or cancellous bone. These preprocessed grafts provide opportunity to customize and precertify the physical form and architectural properties (shape, size, mechanical strength, surface area, porosity) of a graft matrix to an individual site.
The method of sterilization and preparation of allograft tissue has a significant impact on osteoconductive, osteoinductive, and mechanical properties, as well as immunogenicity.124–127 Donor cells and cell fragments are the most immunogenic material in allogenic bone. Processing of allograft bone therefore includes steps that attempt to remove as many cells as possible from the graft. Immunogenicity is further reduced, although not eliminated, by freezing to –20° C.128–136 Freeze-drying is even more effective at reducing the immunogenicity of allogenic bone, but at the price of reducing mechanical strength by 50%.130,137
Using contemporary processing and storage techniques, clinical evidence of overt immunologic reaction against the graft is rare. Even so, histologic evidence of a low-grade inflammatory reaction can be found around essentially all allografts. This reaction probably slows the incorporation of many allografts and may contribute to the failure of some, as suggested by several canine studies that have documented improved biologic behavior in antigen-matched allografts.125,126,137,138 Antigen matching is not currently considered practical in the clinical setting, however. The relatively high current success rates for allografts makes the large cost of antigen matching not feasible and is probably unwarranted in general practice.139
Sterility of frozen allografts is ensured through expedient postmortem harvesting using sterile surgical technique and careful monitoring using surface cultures and recently polymerase chain reaction (PCR) screening for bacterial and viral genome fragments. The current risk of disease transmission via a musculoskeletal allograft is approximately 1 in 1,667,000.140,141 A variety of secondary sterilization procedures have been designed and may be used, depending on the source of the allograft. Ethylene oxide sterilization was evaluated by Cornell,142 who found a 70% decrease in bone induction by demineralized bone powder in rats. Other authors have reported variable changes in inductive capacity of ethylene oxide sterilized matrix.143–145 Heating or autoclaving bone tissue is generally avoided due to their disruption of matrix proteins. Some processing techniques such as high-dose irradiation compromise both the biologic potential of bone matrix, reducing bone formation and union rates,146,147 and also alter the mechanical properties of the graft.148–155 For example, irradiation to 2.5 megarads or freeze-dried processing may reduce the torsional strength of the cortical allograft by as much as 50%.137
Results from other clinical and experimental studies using allograft bone alone in spinal fusions have been mixed. Some investigators have found allograft to be significantly inferior to autogenous bone grafting when compared with other distinctive preparation methods,36,38,149,156–167 whereas others find little or no difference between them.36,62,168–182 Allograft bone appears to be particularly valuable in settings that require the graft to serve a significant mechanical function such as struts or ring allografts for anterior lumbar interbody fusions183–187 or as struts or bone-wire fixation constructs in the upper cervical spine.188–197 In these settings, allografts have essentially replaced the use of autografts from rib, fibula, tricortical iliac crest, and tibial hemicortex grafts, which are all associated with significant donor site morbidity.
In the past decade options for structural materials that can be incorporated into interbody fusion sites have expanded to include the use of structural cages composed of titanium or carbon fiber.197–205 These materials are capable of providing the structural function that is necessary to maintain the height and stability of the interbody site. These cages and wedges lack some of the osteoconductive capacity of allograft bone and the capacity of allograft bone to be biologically incorporated into the fusion mass and remodeled, remaining in the site essentially as a permanent foreign body. Metal cages also make radiographic assessment of the fusion site more difficult. However, in comparison with allograft, metal cages do offer the advantage of more consistent material properties, specifically strength and fracture resistance.
Regardless of material used for structural support, there is relatively general consensus that in order to achieve optimal rates of spinal fusion, the environment within and around these structural allografts or cages should be further supplemented with other osteogenic, osteoconductive, and/or osteoinductive graft materials. Autogenous cancellous bone, bone marrow aspirate,206 processed bone marrow–derived cells,114 processed nonstructural allograft materials, and BMPs3,207,208 are most commonly considered.
Demineralized Allograft Bone Matrix
The history of demineralization as a means to enhance allograft performance is richly linked to many of the recent biologic insights into bone biology and bone healing. It was more than a century ago, in 1889, that Senn reported the repair of long bone and cranial defects in patients with chronic osteomyelitis using hydrochloric acid-treated decalcified heterologous bone implants.66 Although his primary motive was to promote antisepsis within the bone cavities, Senn observed rapid substitution of the demineralized tissue with new bone formation invading from the perimeter of the defects. However, several of Senn’s contemporaries obtained equivocal results, and clinical efforts over the next 70 years were minimal.188,209–211
Reddi and Huggins212,213 revived this concept when they reported on their observation that matrix induced the bone induction phenomenon in rats. Urist went on to demonstrate bone induction using a variety of demineralized matrix preparations in muscular pouches of rabbits, rats, mice, and guinea pigs.214 Subsequently, matrix-induced heterotopic bone formation was documented at many soft tissue sites including muscle, tendon, and fascia,181,213,315-219 as well as in the thymus71 and soft connective tissue of visceral organs.220 Nathanson also observed the differentiation of neonatal embryonic skeletal tissue into cartilage when cultured on demineralized bone matrix substratum and suggested that the tissue transformation of bone induction was analogous to embryonic bone tissue differentiation.221,222
Reddi subsequently characterized the inductive phenomenon of bone matrix as a cascade of events parallel to those occurring in endochondral ossification and postulated that the process was the result of stimulation by a series of soluble matrix factors that potentiated events along the cascade.223–225 In this paradigm, bioactive factors in bone matrix stimulate activation and migration of osteogenic stem cells and progenitor cells. Mitogenic factors promote cell proliferation. Angiogenic factors promote local revascularization, and osteoinductive factors promote osteoblastic differentiation. Subsequently, bone matrix has been shown to contain a rich variety of growth factors and other bioactive molecules in concentrations that are bioactive.226,227
Sato and Urist228 showed that demineralized bone matrix was both inductive and was synergistic with bone marrow–derived cells in healing of rat femoral defects. He went on to provide a clinical outlet for these discoveries by developing a “chemosterilized, autolyzed, antigen-extracted allogenic bone (AAA),” prepared using chloroform-methanol extraction, 0.6 N hydrochloric acid extraction of soluble proteins with partial demineralization, and neutral phosphate autodigestion.215–217219 This preparation appeared to reduce the immunogenicity of the allograft matrix without loss of inductive properties. Using this preparation, Urist reported on 40 patients undergoing posterolateral lumbar spinal fusion with an 80% success rate and a pseudoarthrosis rate of 12%.229
The value of a variety of demineralized bone matrix preparations (chips, fibers, powders) has subsequently been described in a number of settings by a series of authors. Glowacki and colleagues230–232 were among the first to report successful repair of craniofacial defects. Tiedeman and colleagues89 and Wilkins and colleagues233 both reported clinical efficacy in long bone defects. Other reports have also shown efficacy for demineralized bone matrix in spine fusion models.234,235 Some studies have reported a benefit of adding demineralized bone matrix to autograft or ceramic matrices in animal spine fusion models.87,236–240 There is also recent evidence that some demineralized bone matrix preparations can be used as a substrate for selective attachment and concentration of bone marrow–derived osteogenic stem cells and progenitors.113,114
Of recent clinical concern is evidence that suggests that the biologic efficacy of commercially available preparations of demineralized bone matrix materials can vary significantly, depending on the method of processing, the individual batch of bone that is processed, or the donor of the bone that is used. This variation has raised questions about the possible value of implementing generalized standards for either in vitro or in vivo biologic assays for bone from each batch and donor in order to limit the potential for biologically deficient materials from compromising the performance of clinical grafting procedures.123,241,242 At present, without evidence that failed graft procedures cluster around individual donors or batches of demineralized bone, and that these failures can be predicted by any of the available assays, the clinical value and cost effectiveness of biologic assays is uncertain. Regardless, some providers of demineralized bone have elected to implement some form of bioassay and use these data to exclude some bone from use and to make claims of superior or more reliable performance in the marketplace.
Deproteinated Heterologous Bone
In contrast to allograft bone, heterologous bone (xenograft) fails to induce osseous repair due to its high level of antigenicity. Partially deproteinated and partially defatted heterologous bone (Kiel bone or Oswestry bone) does exhibit greatly reduced antigenicity and therefore evokes a minimal immune response.243 The denaturing process, however, also destroys osteoinductive matrix proteins. Accordingly, implantation of such materials in bone defects and muscular compartments has failed to generate bone formation.96
The impregnation of this material with cells capable of osteogenic activity, however, has been studied. Salama and colleagues96 and Plank and colleagues244 demonstrated that deproteinated xenograft bone supplemented with autologous marrow assisted osteogenesis in both experimental animals and humans. Deproteinated bone, in these experiments, served as an osteoconductive scaffolding, providing a stable mechanical environment for revascularization and proliferation and differentiation of osteogenic cells. Salama and Weissman97 reported satisfactory results in clinical attempts to use composite xenograft/autograft (Kiel bone/marrow) in a variety of bone defects. More recently, Rawlinson and colleagues245 reported poor results using bovine-derived Cloward grafts. Due to the wide availability of more effective allograft matrix materials in the United States at similar costs, xenograft materials are not currently used.
Synthetic Bone Graft Materials
Recent years have seen an explosion of new information about the cellular and molecular events involved in the bone healing response (Table 67–1). Purified human recombinant growth factors are now becoming available. Many are active in multiple events in the bone healing process and are therefore candidates as potential therapeutic agents. In addition, rapid developments in porous ceramic materials and bioerodible polymers of biologic and synthetic polymers enable the design of customized matrix materials that can be used both as osteoconductive scaffolds and as delivery systems for bioactive molecules. These converging events are now producing an army of first-generation and second-generation biosynthetic bone grafting materials. It is beyond the scope of this chapter to comprehensively review any one of these areas. Several recent reviews are available.41,124,246,247 The following is intended as an overview of some of the ongoing developments in this area, specifically the application of growth factors, collagen matrices, and ceramics in synthetic bone grafting materials.
Bone Morphogenic Proteins
A major advance occurred in 1978, when Urist and colleagues248 reported the isolation of a hydrophobic, low-molecular-weight protein fraction from insoluble bone matrix gelatin that was responsible for osteoinductive activity.218 Further characterization of this inductive factor, BMP, was made possible by quantitative extraction accomplished by differential precipitation in a buffer containing 4M guanidine hydrochloride.249 Lovell and Dawson went on to report the success of a partly purified BMP preparation on polylactic acid strips in a canine segmental spinal fusion model.250
After an extensive search for the protein responsible for the inductive activity of bone matrix extract, Wozney and colleagues,251 in 1988, identified and characterized three proteins isolated from a highly purified preparation from bovine bone, each capable of inducing bone formation in a rat subcutaneous bioassay. Human cDNA clones for each peptide were isolated and expressed as recombinant human proteins. Two of the encoded proteins were homologous and described as members of the TGF-β super gene family, whereas the third appeared to be a novel polypeptide (BMP-1). BMP-1 has turned out not to be a growth factor at all. Rather, this molecule has been characterized as a procollagen C-proteinase, which may have a biologic function in the activation of TGF-β-like molecules including the BMPs.252,253
The BMP story has developed even more rapidly in recent years. At the time of this writing the BMP family of growth factors currently includes 14 members. BMP-2 through BMP-14 are homologous proteins with molecular weight of 12 to 14 kD that are post-transcriptionally modified by glycosylation and are secreted as homodimers or heterodimers of 110 to 140 amino acid peptides linked by one disulfide bond (≈30 kD).251,254,255 In vivo, these proteins are secreted as soluble factors that have autocrine and paracrine effects. BMP-7 (OP-1) can also be found in systemic circulation and may also have positive hormonal effects on kidney function.256,257 BMPs are also embedded in bone matrix, both as homodimers and as heterodimers at a concentration of roughly 1 mg/kg of bone, where they are believed to play a role in bone remodeling and the coupling of osteoclastic and osteoblastic activity. Of interest is the finding that as much as 65% of the BMP in bone matrix is BMP-3, which is less active in bone formation and may, in fact, be a negative regulator of bone formation.258,259
Figure 67–2 summarizes these proteins along with synonyms or alternative names that are now, or have been, used for some of these molecules. Figure 67–3 illustrates the percent RNA sequence homology within and between subgroups of the BMP protein family. Each of these proteins can interact with one or more of a family of cell surface receptors. Cells must express both type I and type II receptors (serine/threonine kinases) in order to be responsive to BMPs because a type I and type II receptor must interact in the presence of a BMP to mediate a cellular response. To date, three type I and three type II BMP receptors have been identified. BMPR-IA binds only BMPs. BMPR-IB binds BMPs and müllerian inhibitory substance (MIS). ALK-2 (also a type I receptor) binds BMPs and activin. BMPR-II binds only BMPs, and the activin type II receptors, ActR-IIA, and ActR-IIB bind both BMPs and activin.260 In vitro, BMPs demonstrate dose-responsive modulation in responsive cells, both primary osteogenic cells and in cell lines, in the range of 1 to 100 ng/mL, but each BMP demonstrates a unique and variable binding pattern for the individual receptors, as illustrated in Figure 67–4. A great deal of functional redundancy and promiscuity between these proteins and receptors appears to be present, though these genes appear to be independently regulated in both space and time in embryonic development and in bone healing settings, and expression of specific inhibitors of BMP function (e.g., noggin, chordin, connective tissue growth factor, follistatin) also plays a role.254,261–266
Among the BMP homodimers that are most active in bone induction in vivo are BMP-2, BMP-4, BMP-6, BMP-7 (i.e., Osteogenic Protein-1 or OP-1), and BMP-9. BMP-2 and BMP-7 have both been developed for clinical applications in bone grafting and skeletal reconstruction. BMP-13 (i.e., MP53 or GDF-5) and BMP-14 (i.e., GDF-6) are also under development.267–269 At present, both BMP-2 and OP-1 (BMP-7) have been fully evaluated and approved by the FDA for use in spinal fusion. This approval is specifically limited to the use of BMP-2 in a collagen carrier (INFUSE, Medtronic Sofamore Danek, Minneapolis) in the setting of anterior interbody fusion using a metallic cage. OP-1 Putty (Stryker, Kalamazoo, Mich.) can be used in patients who have failed a previous spinal fusion surgery and are not able to provide their own bone or bone marrow for grafting because of a condition such as osteoporosis, diabetes, or smoking.
A large number of animal studies have demonstrated the promise and relative safety for these proteins as powerful stimulants of a local bone healing response in rodents, sheep, canines, and nonhuman primates, using various carrier matrices and a dosage range of 100 to 10,000 µg/mL.255,270–295 Schimandle and colleagues293 reported 100% union in an uninstrumented posterolateral intertransverse fusion model in the rabbit using BMP-2 delivered in a collagen carrier, compared with only 42% fusion with autogenous corticocancellous iliac crest bone. Muschler and colleagues,296 using an instrumented posterior canine spinal fusion model, found that BMP-2 delivered in a degradable polymer carrier (PLGA) had comparable efficacy to autogenous cancellous bone. Cook and colleagues297 found similar results in a canine spine model using OP-1 (i.e., BMP-7). The use of rhBMP-6 stOPCs in a carrier of guanidine-extracted demineralized (gDBM) bone matrix significantly enhanced the rate and strength of single-level posterolateral spinal arthrodeses in the New Zealand white rabbit, compared with iliac crest bone graft, gDBM, and decortication alone.298 Using a rabbit model, Fu and colleagues demonstrated enhancement of posterolateral lumbar spine fusion using low-dose rhBMP-2 and cultured marrow stromal cells.
Several prospective clinical trials evaluating BMPs in the setting of spinal fusion have been performed, generally reporting performance that was comparable with autogenous cancellous bone, with fusion rates between 80% and 99%.299–305 These initial studies strongly support the clinical value of BMPs, particularly BMP-2, which has been most thoroughly studied in the clinical setting to date.
In a prospective, randomized, controlled, multicenter clinical pilot study, Vaccaro and colleagues306 demonstrated that the rates of radiographic fusion, clinical improvement, and overall success associated with the use of OP-1 Putty were at least comparable with that of the autograft controls for at least 48 months after surgery. Singh and colleagues307 performed a prospective, single-institution, clinical case-matched, radiographic cohort study involving 52 patients who underwent posterolateral lumbar arthrodesis with pedicle screw instrumentation. Using thin-slice computed tomography (CT) analysis, the authors demonstrated 97% fusion rates in the rhBMP-2 group compared with 77% in the iliac crest bone graft at 2 years. The authors concluded that the adjunctive use of rhBMP-2 and iliac crest bone graft seems to be safe and results in significantly larger and more consistent posterolateral fusion masses.307 In a human posterolateral lumbar spine trial, OP-1 reliably induced viable amounts of new bone formation, but the fusion success rate evaluated by surgical exploration was only 4 of 7.308 Lewandrowski and colleagues309 reported vertebral osteolysis with the use of rh-BMP-2 in posterior lumbar interbody fusions with 5 out of 68 patients developing osteolysis within 4 months of surgery. Violation of the endplate during decortication was thought to be a contributing factor. This often resolves spontaneously. Similar observations were made in the cervical spine by Vaidya and colleagues.310 In a prospective, consecutive patient enrollment with a minimum 24-month follow-up, 30 patients underwent anterior interbody allografts alone and 45 patients underwent anterior interbody allograft filled with rhBMP-2. All cases had posterior pedicle screw instrumentation. A total of 165 surgical levels (62 allograft alone; 103 allograft + BMP) were included. In the allograft rhBMP-2 group, fusion rates were 94%, 100%, and 100% at 6, 12, and 24 months, respectively, after surgery, while in the allograft-only group, fusion rates were 66%, 84%, and 89% at the same time intervals. Clinical outcomes were significantly improved in rhBMP-2 group when compared with the allograft group at 6 months. There were no revisions in the rhBMP-2 group and four revision fusion surgeries (13%) in the allograft group.311 In a randomized, controlled trial in patients older than 60 years of age, Glassman and colleagues312 concluded that RhBMP-2/INFUSE is a viable iliac crest bone graft replacement in older patients in terms of safety, clinical efficacy, and cost-effectiveness.
Recently, several investigators have evaluated the use of BMPs in cervical spine surgery.310,313,314 Anterior cervical discectomy and fusion performed with rhBMP-2 (0.9 mg BMP per level) allograft was found to be as effective as iliac bone graft in terms of patient outcomes and fusion rates. Safety concerns related to neck swelling and higher initial costs were associated with patients in the bone morphogenic protein group.313 Vaidya and colleagues compared 22 patients treated with rhBMP-2 and PEEK cages with 24 in whom allograft spacers and demineralized bone matrix was used. Radiographic examination following surgery revealed end plate resorption in all patients in whom rhBMP-2 was used. This was followed by a period of new bone formation commencing at 6 weeks. In contrast, allograft patients showed a progressive blurring of endplate-allograft junction. Dysphagia was a common complication and it was significantly more frequent and more severe in patients in whom rhBMP-2 was used. Postoperative swelling anterior to the vertebral body on lateral cervical spine radiograph was significantly larger in the rhBMP-2 group when measured from 1 to 6 weeks after which it was similar. There was no significant difference in the clinical outcome of patients in the two groups at 2 years. The authors concluded that despite providing consistently good fusion rates, they have abandoned using rhBMP-2 and PEEK cages for anterior cervical fusion, due to the side effects, high cost, and the availability of a suitable alternative.315 Shields and colleagues316 reviewed 151 patients who underwent either an anterior cervical discectomy and fusion (n = 138) or anterior cervical vertebrectomy and fusion (n = 13) augmented with high-dose INFUSE (BMP-2; Medtronic Sofamor Danek). They found a high morbidity rate with a total of 35 (23.2%) patients having complications that the authors thought were attributable to the use of high-dose INFUSE in the cervical spine. Fifteen patients were diagnosed with a hematoma including 11 on postoperative day 4 or 5, of whom 8 were surgically evacuated. Thirteen individuals had either a prolonged hospital stay (>48 hours) or hospital readmission because of swallowing/breathing difficulties or dramatic swelling without hematoma. The authors concluded that putative inflammatory effect that contributes to the effectiveness of INFUSE (Medtronic Sofamor Danek) in inducing fusion may spread to adjacent critical structures and lead to increased postoperative morbidity.316 On the basis of their experience with 69 patients who underwent anterior cervical fusion, Smucker and colleagues317 concluded that use of rhBMP-2 in the anterior cervical spine is associated with an increased rate of clinically relevant swelling events.
One of the principle requirements for optimal BMP activity is the presence of a local population of target cells, most likely osteogenic stem cells and progenitor cells that are responsive to the protein (i.e., they express appropriate receptors). In order for a BMP to be optimally effective, these target cells must be both available and activated in sufficient numbers to produce the desired result. If an optimal number of responsive cells is not present within the tissue volume that is exposed to the protein following implantation, the biologic response to the protein will inevitably be reduced and the implantation of a BMP may be completely ineffective. Variation in the concentration or biologic potential of target cell populations in bone, bone marrow, periosteum, and other tissues (e.g., muscle, fat) may explain much of the apparent variation in the magnitude and type of response seen to BMPs and other growth factors from site to site and individual to individual.99,100,318
Preclinical evaluation of BMPs in a series of animal models from rats to rabbits to dogs to nonhuman primates demonstrated the need for delivery of dramatically high concentrations of BMP to graft sites in higher animals.274 In fact, the formulations of BMP that are currently available deliver an amount of BMP that is roughly 50 times greater than the total amount of BMP that is present in an entire human skeleton. INFUSE (Medtronic Sofamor Danek) delivers BMP-2 in solution at a concentration of 1.5 mg/mL to be combined with a collagen carrier, resulting in a implanted concentration slightly less than 1 mg/mL. Similarly, the OP-1 device delivers 3.5 mg of OP-1 in a final volume of approximately 4 mL. The reason for this escalation of dose has not been clearly established, though several factors are likely to contribute. Species-specific differences in dose response may exist at the target cell level, though this has not been a consistent finding in in vitro culture of primary osteogenic cells. However, individual species do demonstrate significant differences in the concentration and prevalence of responsive target cells in local tissues and consistently lower numbers in higher animals. The geometry involved in mating active BMP with a responsive target population of cells in larger graft sites may also contribute. For both of these reasons, a huge dose may be necessary to provide a burst of BMP delivery that is sufficiently large that the BMP diffusing away from the graft site will penetrate into regional tissues to a sufficient depth at a sufficiently large concentration to activate enough stem cells and progenitors. As the graft sites become larger, activation of more target cells is required. This results in the need for a greater degree of penetration that can only be achieved with a larger dose. Similarly, if the concentration and prevalence of target cells in regional tissues decrease, as they do in higher animals, activation of a similar number of cells will require even deeper tissue penetration. These factors likely contribute to the seemingly exponential increase in dose that is necessary in larger animals.41 A second possible explanation for the massive dose relates to the issue of BMP retention at the graft site. A massive initial dose may also ensure that a sufficient, though perhaps small, quantity of BMP will remain at the site long enough to result in activation of target cells that may not enter the graft site until several days after implantation, with the associated inflammatory response or angiogenic response following the surgical trauma.
These issues in BMP delivery and function in a graft site suggest a number of options that could be used to increase the exposure of BMP to an appropriate target population of cells, thereby improving its performance. Direct delivery or supplementation of the target cell population in the graft site is one option. Evidence is provided by a number of studies that addition of a target population (e.g., bone marrow–derived cells) to a site of BMP implantation will significantly improve bone healing.92,318 Another possible method for enhancing the performance of BMPs is to refine the method and rate of BMP delivery into the graft site. The chemical surface of the matrix may influence the protein binding, its conformation, and stability. In some cases, BMP binding to matrix can actually enhance the biologic performance.319–321 The four major categories of BMP carrier materials are natural polymers such as collagen, hyaluronans, fibrin, chitosan, silk, alginate, and agarose; inorganic materials such as low- and high-temperature calcium orthophosphates (calcium phosphate cements and sintered ceramics) and calcium sulphate cements; poly(α-hydroxy acid) synthetic polymers such as PLA, polyglycolide (PLG); and their copolymers (poly[D,L-lactide-co-glycolide]) (PLGA).322 Furthermore, the carrier (collagen, ceramic, polymer) may have its own biologic effects associated with the release of ions or other degradation products.41 Currently both the OP-1/BMP-7 (Osteogenic Protein-1/BMP-7; Stryker) and INFUSE (Medtronic Sofamor Danek) use type I bovine collagen as the carrier. In the case of OP-1, the protein is lyophilized onto the surface of the collagen. With INFUSE, it is adsorbed onto the surface of the collagen out of an aqueous solution. Other options include release from degradable polymers, liposomes, and collagen-hydroxyapatite microsphere.323–325
Yet another option for enhancing the performance of BMPs is to prolong the period of time that effective concentrations of the protein are present in the graft site. Residence time of bioactive protein in a graft site is a complex function of the rate of delivery (e.g., release or solubilization); the rate of consumption within the graft site (e.g., degradation, inactivation, binding to inhibitors); and the rate of clearance from the graft site (e.g., diffusion, convection). With BMP preparations currently available, release kinetics are relatively rapid. Pharmacokinetic studies of residence time for OP-1 delivered as a lyophilized protein from a collagen carrier in a rabbit model indicate that the protein is released into the site and retained in measurable concentrations for approximately 7 days, with a maximal release rate in the first 24 hours. The implanted rhOP-1 diffuses out of the immediate graft site at low concentrations, entering systemic circulation, where it is rapidly cleared. Approximately 50% of the implanted dose is excreted in the urine.326 In the case of BMP-2 in the INFUSE Device, release kinetics are similar, with 50% of the rhBMP-2 cleared from the site in 48 hours and less than 1% remaining at 2 weeks.327
Longer residence time within the site is associated with improved efficacy at lower protein concentration.328 Residence time is influenced by the affinity of the protein to the carrier.329,330 Residence time can also be increased by modifying the BMP protein itself to reduce its solubility and therefore its rate of diffusion out of the implant.331 Prolonged residence time within the graft site may have two effects. It may allow the initial gradient of protein concentration around the site to be maintained for a longer period, which may positively influence chemotactic effects that may be mediated by the protein, drawing more activated progenitors and other cells into the defect. Prolonged residence may also serve to maintain a functional concentration of protein in the graft site for a longer time, providing the opportunity for additional stem cells and progenitors to migrate into the graft site where they may become activated.
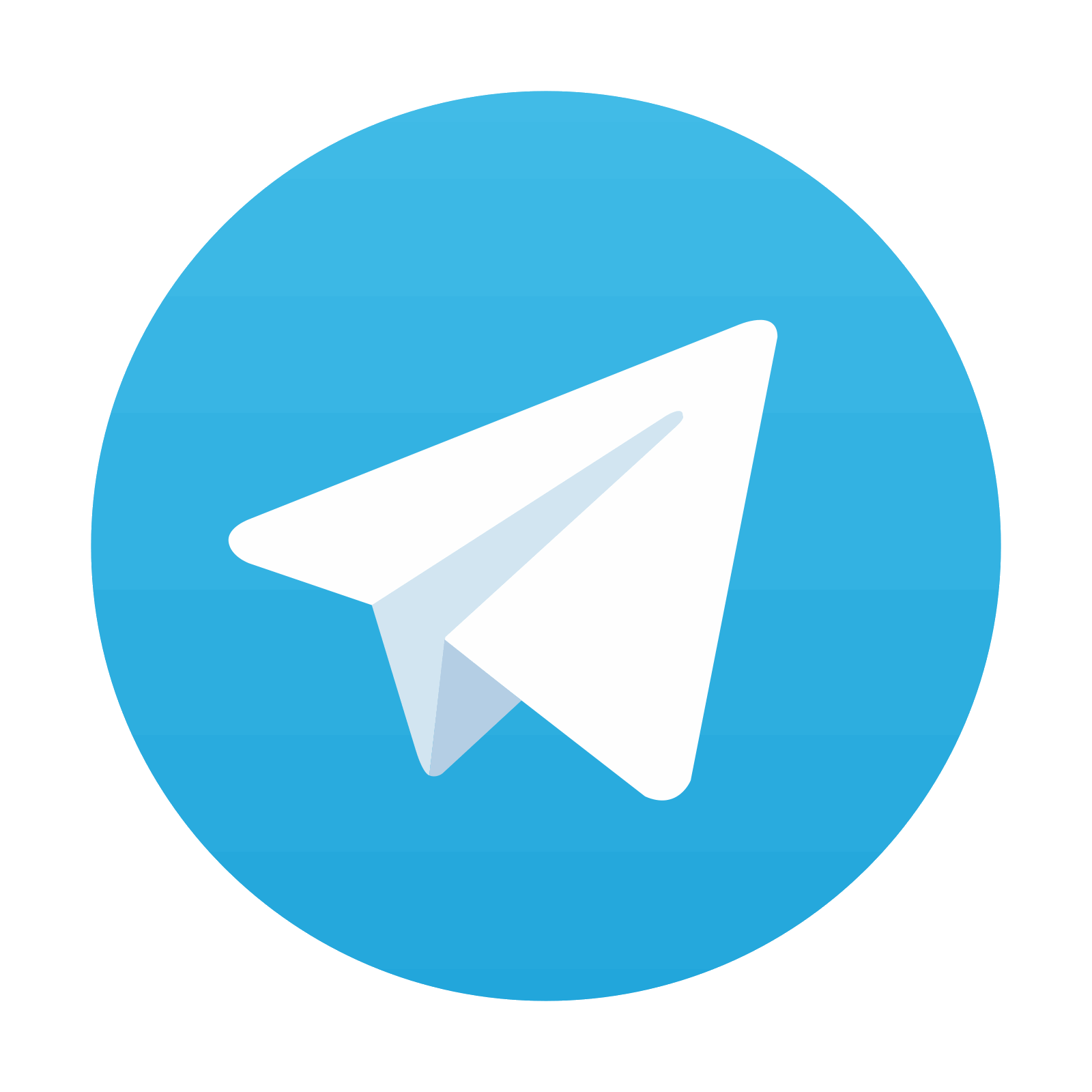
Stay updated, free articles. Join our Telegram channel
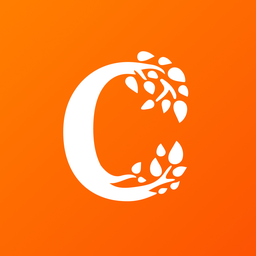
Full access? Get Clinical Tree
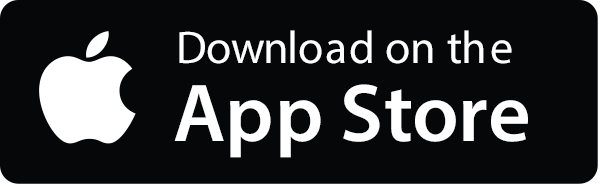
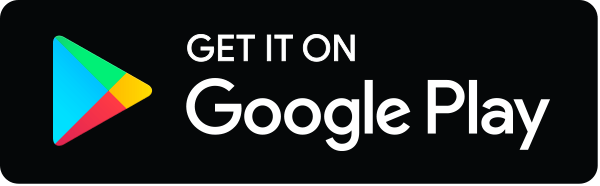