Fig. 53.1
Schematic drawing of the different zones of articular cartilage and subchondral bone
Subchondral bone is a complex tissue consisting of water, collagen type I, and hydroxyapatite, with the two latter components providing the tissue’s stiffness and compressive strength [14, 15, 17]. The compressive modulus of subchondral bone is higher than that of cartilage. The different morphological compositions and mechanical properties of subchondral bone and cartilage indicate the complexity of the tissue interface.
The osteochondral interface is described by the interaction of calcified cartilage and the underlying subchondral bone [18]. Structurally, collagen fibers extend from the deep zone to calcified cartilage through a wavy tidemark, which enables the dispersal of force through the vertical orientation of collagen fibrils [19]. However, despite the fact that calcified cartilage is mineralized tissue, its mechanical strength is lower than that of the subchondral bone [20]. Calcified cartilage is interdigitated with subchondral bone, but fibers do not extend across the zone into the bone [19, 21]. The wavy tidemark and vertically oriented fibers at the tidemark, as well as interdigitations present at the interface, may allow for reducing stress concentrations, as well as better integration with the underlying subchondral bone [14, 19].
Osteoarthritic degenerative changes, such as articular cartilage loss, subchondral bone thickening, and osteophyte formation, may be developed, triggered by a multitude of factors including aging, trauma, obesity, mechanical overload, congenital disorder, and infection [22–26]. The primary morphologic changes include thinning, fissuring, and fragmentation of articular cartilage. With progression of the disease comes a continuous loss of articular cartilage, accompanied with the decrease of collagen type II and aggrecan [27, 28], leading to exposure of subchondral bone. Secondary changes are frequently seen in the underlying bone, such as sclerosis, cystic change, and new bone formation (Fig. 53.2).


Fig. 53.2
Radiography of osteoarthritic knee joint. In osteoarthritis, the loss of cartilage (joint space narrowing) and subchondral bone change such as sclerosis, cystic change, and new bone formation (osteophyte) are frequently seen (arrows)
53.3 Strategy for Osteochondral Repair
For an ideal repair of osteochondral lesions, it is important to regenerate subchondral bone and to facilitate zonal restoration of cartilage and subchondral bone, layer by layer, mimicking the natural articular structure [11, 29–34]. As a strategy to regenerate these structures in a layer-by-layer fashion, biphasic or triphasic constructs have been developed due to both mechanical and biological reasons, including the acquisition of initial mechanical strength, mimicking a natural articulate structure, a uniform tidemark at the osteochondral junction, and integration of the biphasic implant with host tissue to sustain biological function [9, 35–44]. For satisfying the biological requirements, an osteochondral implant should ideally have a rigid osseous layer (to support the overlying cartilage and integrate with the native bone) and a chondral layer (to allow the seeding and proliferation of chondrocytes or mesenchymal stem cells (MSCs) and subsequent deposition of cartilaginous ECM).
53.4 Choice of Cells
The most direct cell source may be the biopsy specimens taken from the patients, from which mature osteoblasts and chondrocytes may be obtained. However, as the number of cells obtained is usually limited, it is typically not enough to allow seeding onto the scaffolds. Also, the expansion of primary cells may result in a loss of differentiation capacity; for example, the expansion of articular chondrocytes can lead to dedifferentiation into fibroblast [45–47]. To overcome such potential problems with respect to dedifferentiation, a three-dimensional (3D) culture can be used to retain the cellular phenotype and avoid dedifferentiation [48]. The most common method is the use of various scaffolds to produce a 3D culture condition [49, 50] and may be combined with the supplementation of growth factors [51], the use of bioreactor [52], mechanical stimulation of the cells [53, 54], and the use of low oxygen tension [55, 56] during cultivation. Also, even if chondrocytes lose their differentiated phenotype, dedifferentiated chondrocytes can regain their differentiated phenotype through the redifferentiation process of cultivation in a 3D scaffold combined with growth factors [57, 58].
As an additional option, stem cells may represent promising alternatives [59, 60]. Specifically, mesenchymal stem cells (MSCs) have the capability to differentiate into a variety of connective tissue cell types, including bone, cartilage, tendon, muscle, and adipose tissue [10, 61]. These cells may be isolated from various tissues, such as bone marrow, skeletal muscle, synovial membrane, adipose tissue, and umbilical cord blood [5, 6, 61–64]. Moreover, allogeneic MSCs [10, 65] or induced pluripotent stem (iPS) cells [66, 67] may also be considered. However, there have not been much evidence using these cells forth coming in terms of preclinical and clinical safety, and thus further studies with such cells are likely necessary.
53.5 Choice of Materials
Several methods have been proposed to develop biphasic scaffolds with the hybridization of two distinct biomaterials, each of which being adequate to integrate with the respective surrounding tissue [68]. Many specific material types have been developed for both cartilage and bone regeneration, which are typically made of biocompatible and biodegradable polymers. For the cartilage layer, natural or synthetic polymer-based scaffolds are commonly used. On the other hand, for a scaffold of the subchondral bone layer, it is important to choose materials with initial mechanical strength, good bone ingrowth, and integration of native surrounding bone. Ceramics, glasses, and metallic materials are commonly used. Also, natural or synthetic polymers, similar to cartilage layer, could be used alone or combined with ceramics [36, 37, 69–72].
The natural polymers could provide a naturally occurring environment for the cells and tissues and thereby potentially facilitate cell proliferation and differentiation [73, 74]. Moreover, natural polymers usually contain specific molecular domains that can support and guide cells at various stages of their development [14, 68]; thus biological interaction of the scaffold with the host tissue can be enhanced. However, they are, in general, biomechanically weak and less stiff than other materials [14]. As a source of materials, collagen, gelatin, glycosaminoglycan, chitosan, starch, hyaluronic acid, alginate, and bacterial-sourced polymers (hydroxyalkanoates) are commonly used.
Biodegradable synthetic polymers offer several advantages over other materials for developing scaffolds in tissue engineering. The main advantages are being able to control mechanical properties (i.e., strength and stiffness) and degradation speed [75]. Synthetic polymers are also attractive because they can be fabricated into various shapes with a desired pore according to the speed of cell migration or tissue ingrowth [76]. Moreover, the progression of current techniques such as electrospinning methods and the 3D printer have enabled the simple design and fabrication of scaffolds, which mimic the original tissue structure [77–79]. On the other hand, synthetic polymers have limitations in bioactivity due to their hydrophobic surface not supporting cell attachment and proliferation [80–83]. Surface treatment with chondroitin sulfate [84], silicate [85], and alkaline [81] could increase hydrophilicity and provide a suitable scaffold for tissue engineering. As a source of biodegradable synthetic polymers, poly(glycolic acid) (PGA), poly(d,l-lactic-co-glycolic acid) (PLGA), poly(l-lactic acid) (PLLA), poly(caprolactone) (PCL), and poly(ethylene glycol) (PEG) have been commonly used.
Ceramics, such as hydroxyapatite (HA) or other calcium phosphates, such as tricalcium phosphate (TCP), are widely used for bone tissue engineering [86–89]. These materials promote the formation of a bone-like tissue and enhance integration of the scaffold to the host tissue due to excellent osteoconductivity and osteoinductivity. On the other hand, these scaffolds have low structural integrity being brittle and unsuitable for applications under mechanical stress, although they exhibit suitable stiffness [14]. The degradation behavior of these scaffolds can be controlled by changes in the porous structures, which can be tailored in terms of their degradation kinetics appropriate for bone tissue engineering. It is also well known that increasing porosity impairs further the mechanical properties of bioceramic scaffolds. This problem can be solved by modifying any porous scaffolds with infiltration or coating by biodegradable polymers [90–92].
53.6 Current Status and Issues
There have been many therapeutic procedures investigated to biologically repair damaged cartilage, some of which are already at the stage of clinical application. On the contrary, considering the higher incidence of OA, which involves subchondral bone pathology, by comparison to isolated chondral injury [3, 93–97], there is an urgent need to develop novel therapeutic methods for osteochondral repair with clinical relevance. In this regard, the number of animal experiments and clinical trials to treat osteochondral lesions has been recently increased [40, 98–102]. Also, we have originally developed a hybrid implant composed of artificial bone coupled with a mesenchymal stem cell (MSC)-based scaffold-free tissue-engineered construct (TEC) and demonstrated its feasibility for osteochondral repair in a rabbit osteochondral defect model [12, 103]. Our experimental details are described below.
53.7 Our MSC-Based Hybrid Implant for Osteochondral Repair
Currently, artificial bones generated from hydroxyapatite (HA) or beta-tricalcium phosphate (bTCP) have been widely used for clinical treatment of bone defects after fractures or after resection of bone tumors [88, 104, 105]. We have developed a novel fully interconnected HA artificial bone with a sufficient initial strength, as well as an excellent bone formation capacity [88, 89] and previously reported the feasibility of this implant to repair subchondral bone [88]. In addition, we have developed a scaffold-free three-dimensional tissue-engineered construct (TEC) composed of MSCs derived from the synovium and extracellular matrices (ECMs) synthesized by the cells [6] and demonstrated the feasibility of the resultant TEC to facilitate cartilage repair in a large animal model [5, 10]. These TECs are developed without an artificial scaffold, and, thus, their implantation could eliminate or minimize the risk of potential side effects induced by extrinsic chemical or biological materials. Furthermore, such TECs are highly adherent to cartilage matrix, and secure integration of the TEC to adjacent cartilage tissue is observed after implantation. Therefore, we hypothesized that combined constructs of TEC and the fully interconnected HA-based artificial bone would effectively repair an osteochondral lesion and test this hypothesis using a rabbit osteochondral defect model (Fig. 53.3). At 6 months post implantation, osteochondral defects treated with the hybrid implants exhibited more rapid subchondral bone repair coupled with the development of cartilaginous tissue with good tissue integration to the adjacent host cartilage (Fig. 53.4). Conversely, the control group, in which HA alone was implanted into the osteochondral defect, exhibited delayed subchondral bone repair (Fig. 53.4). In addition, the repair cartilaginous tissue in this group had poor integration to adjacent cartilage and contained clustered chondrocytes, suggesting an early OA-like degenerative change at 6 months post implantation. Biomechanically, the osteochondral repair tissue treated with the combined implants at 6 months restored tissue stiffness, similar to normal osteochondral tissue. Therefore, we concluded that the hybrid implants significantly accelerated and improved osteochondral repair [103].



Fig. 53.3
(a) The hybrid implant generated with a tissue-engineered construct (TEC) and an artificial bone. (b) Osteochondral defects in the femoral groove of the rabbit knee. (c) Schematic representation of implantation of TEC and artificial bone. Quoted and modified from [103] (Shimomura et al., Tissue Eng Part A, 2014)

Fig. 53.4
Toluidine blue staining of repair tissues in control (HA alone) and TEC/HA group. Note that the repair tissue in defects treated with the TEC/HA implant sustained good tissue integration to the adjacent host tissue, while that of control group showed poor integration (arrow). Cellular morphology in defects treated with the TEC/HA implant showed round-shaped cells in lacuna, while that in control group showed cell clustering in lacuna. Bar = 1 mm (upper images). Bar = 20 μm (lower images). Quoted and modified from [103] (Shimomura et al., Tissue Eng Part A, 2014)
To further improve the repair quality and shorten the maturation time, additional options for the artificial bone component should be evaluated and compared with outcomes with HA. bTCP is an alternative to consider, as it is a highly biocompatible material that provides a resorbable interlocking network to implants and is resorbed more rapidly than HA in vivo [89]. Therefore, the use of bTCP might be advantageous and may result in more efficient and rapid subchondral bone remodeling after implantation. We hypothesized that a bTCP-based hybrid implant coupled with a synovial MSC-derived TEC would exhibit superior osteochondral repair when compared with an HA-based hybrid implant and test this hypothesis using a rabbit osteochondral defect model mentioned above. Osteochondral defects treated with the TEC/bTCP implants showed more rapid subchondral bone repair at 1 month, but the cartilaginous tissue deteriorated over time out to 6 months post implantation (Fig. 53.5a, b). On the other hand, osteochondral defect treated with TEC/HA showed the delayed subchondral bone repair at 1 month but similar quality of subchondral bone repair to TEC/bTCP at 2 months (Fig. 53.5a, b). Notably, the repair tissue maintained good histological quality out to 6 months after implantation and also exhibited better biomechanical properties at 6 months as compared with the TEC/bTCP implants (Fig. 53.6). Contrary to our hypothesis, the TEC/HA hybrid implant facilitated better osteochondral repair than did the TEC/bTCP implant. The results of the present study suggest the importance of a stable restoration of subchondral bone for long-term effective osteochondral repair rather than rapid remodeling of subchondral bone.





Fig. 53.5
(a) Hematoxylin and eosin (H&E) staining of repair tissue resulting from the implantation of TEC/HA or TEC/bTCP hybrid implants. The osteochondral defect treated with TEC/bTCP showed rapid subchondral bone repair at 1 month. The osteochondral defect treated with TEC/HA showed the delayed subchondral bone repair at 1 month but similar quality of subchondral bone repair to TEC/bTCP at 2 months. Bar = 1 mm. (b) Toluidine blue staining of repair tissue resulting from the implantation of TEC/HA or TEC/bTCP hybrid implants at 6 months after surgery. The repair tissue exhibited fibrocartilaginous-like tissue with weak toluidine blue staining in the TEC/bTCP group, while that did in hyaline cartilage-like features, and the chondrocytes were arranged in longitudinal columns in TEC/HA group. Bar = 1 mm (upper images). Bar = 20 μm (lower images). Quoted and modified from [12] (Shimomura et al., Am J Sports Med, 2017)

Fig. 53.6
The cartilage tissue stiffness of a healthy rabbit and after implantation of a TEC/HA or TEC/bTCP hybrid implant at 6 months after surgery. The TEC/HA hybrid implant exhibited enhanced mechanical properties compared with the TEC/bTCP hybrid implant. *p < 0.05. Quoted and modified from [12] (Shimomura et al., Am J Sports Med, 2017)
53.8 Future Directions
The management of OA remains challenging and controversial. Considering the steady progression of tissue engineering and cell-based technologies over the past decade, we may have new therapeutic options for osteochondral repair in clinical practice. In this chapter, we have focused on the current techniques and recent advances of tissue-engineered biomaterial scaffolds as well as our novel MSC-based hybrid implant for the treatment of osteochondral lesion. There have been many promising scaffolds developed, some of which contribute to good osteochondral repair in vivo. In addition, the recent work has been focused on not only investigating the effectiveness of materials or cells but also to apply several new concepts and techniques such as mechanical [100], microstructural [76], and local microenvironment modification [106] for the design and fabrication of scaffolds. Therefore, the application of additional new implants to osteochondral lesions could be expected in the near future. On the other hand, the most suitable biomaterials for the cartilage or subchondral bone layers have not been fully investigated, while there are many biomaterials available for osteochondral repair. Therefore, the comparison of these materials should be performed to ultimately determine the ideal material. Further studies will be needed and should be conducted in a methodologically rigorous fashion.
Acknowledgments
This study was supported by a grant from the New Energy and Industrial Technology Development Organization, Japan, and Grant-in-Aid for Scientific Research (B), Japan Society for the promotion of Science, Japan.
References
1.
Harris Jr ED. The bone and joint decade: a catalyst for progress. Arthritis Rheum. 2001;44(9):1969–70.PubMed
2.
3.
4.
5.
Ando W, Tateishi K, Hart DA, et al. Cartilage repair using an in vitro generated scaffold-free tissue-engineered construct derived from porcine synovial mesenchymal stem cells. Biomaterials. 2007;28(36):5462–70.
6.
7.
8.
9.
Kandel RA, Grynpas M, Pilliar R, et al. Repair of osteochondral defects with biphasic cartilage-calcium polyphosphate constructs in a sheep model. Biomaterials. 2006;27(22):4120–31.CrossRefPubMed
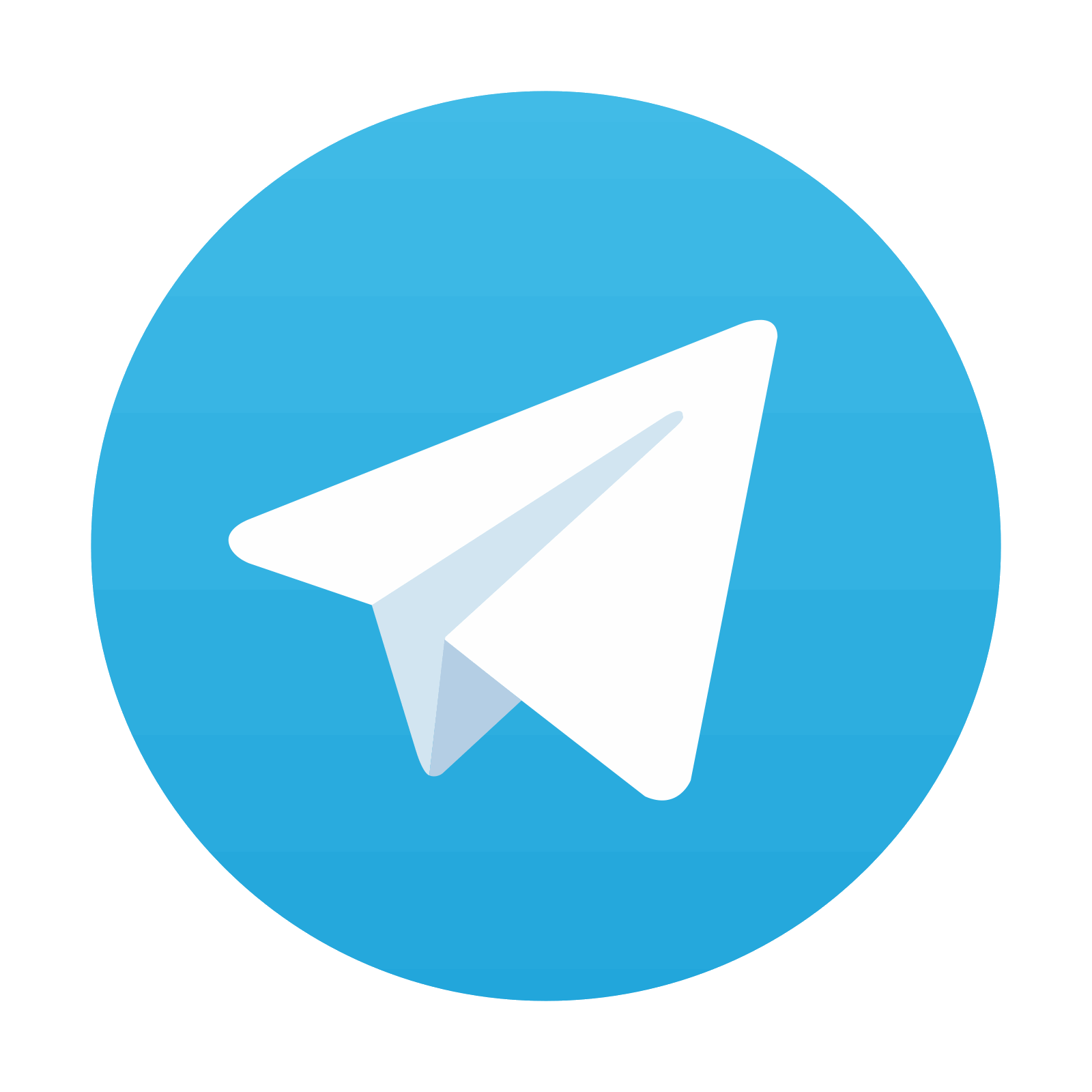
Stay updated, free articles. Join our Telegram channel
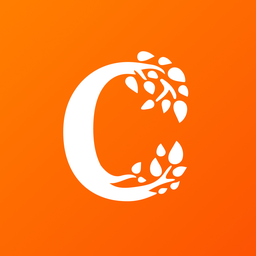
Full access? Get Clinical Tree
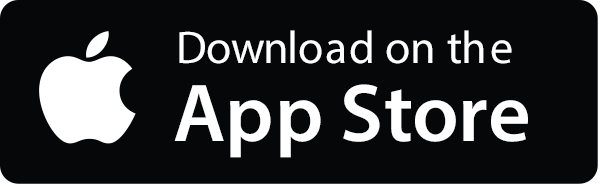
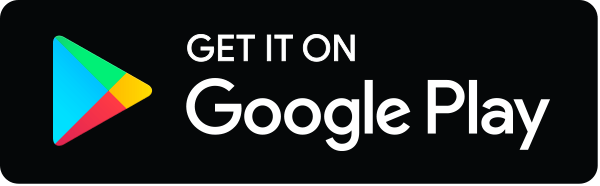