Chapter 16 Mechanisms of Tissue Damage—Free Radicals and Fibrosis
Systemic lupus erythematosus (SLE) is a chronic, complex inflammatory autoimmune disease involving multisystem manifestations. Diverse autoantibody response, directed against a multitude of self-antigens, is a characteristic of the disease. The targets of these antibodies are localized in the nucleus, cytoplasm, or cell membranes. SLE it thought to arise as a consequence of genetic and environmental factors.1 To date, multiple mechanisms of tissue damage have been implicated in SLE, including oxidative stress and free radicals, fibrosis, complement-mediated pathways, and a panoply of enzymatic cascades including matrix metalloproteinases, the renin-angiotensin system, plasmin, and kallikreins. This review focuses on two of these mechanisms: free radicals and oxidative stress and fibrosis.
Free Radicals and Oxidative Stress
Free radicals (reactive oxygen species, oxygen-based free radicals, reactive nitrogen species, and nitrogen-based free radicals), free radical–mediated oxidative damage, and its natural corollary—namely, oxidative modification of proteins—are seen in SLE.2–10 That oxidative stress, through the process of lipid peroxidation and resulting products of oxidative damage, may be implicated in the pathogenesis of SLE is evidenced by (1) an enhanced urinary excretion of isoprostanes, a well-established index of lipid peroxidation, in patients with SLE; (2) the fact that lipid peroxidation–derived short-chain aldehyde levels are significantly elevated in children with high SLE disease activity; and (3) the finding of oxidized LDL elevations along with increased levels of autoantibodies against oxidized LDL in women with SLE. The involvement of free radical–mediated lipid peroxidation is also suggested by the observation that lipid peroxidation–specific epitopes are detected in tissues from the patients.11
Free Radicals, Antioxidant Enzymes, and Lipid Peroxidation
Compared with most anaerobic organisms, aerobes have an efficient metabolism owing to the high reduction potential of molecular oxygen, which acts as the terminal electron acceptor for respiration. However, because both chemical reduction and metabolic reduction of oxygen result in the production of highly toxic free radicals, this advantage comes with a price.12
Detection of Radicals
Free radicals possess unique physical properties that permit their detection and analysis. These properties derive from the fact that any charged particle that is spinning generates a magnetic field. Therefore, electrons and protons create a weak magnetic field. Paired electrons occupying an orbital, owing to their opposing spins, cancel each other’s magnetic fields. On the other hand, a free radical can be detected and analyzed by means of electron paramagnetic resonance spectroscopy because free radicals have a weak magnetic field as a result of the unopposed electron.13
Radical Chemistry—A Brief Outline
A free radical state can be induced by physical means such as irradiation with x rays or ultraviolet (UV) light or by chemical means such as with compounds known as initiators. Most importantly, substances attain an unusual chemistry and molecular configurational changes once they become free radicals. Substances change their physical-chemical properties and shapes considerably as free radicals. Free radicals blaze their own patterns of chemical reactions. In order to distinguish them from the normal organic reactions, such chemical reactions are sometimes referred to as anti-Markownikoff or Kharasch mechanisms. The important aspect regarding radical chemistry is the altered function of molecules involved consequent to the altered size and shape.13
Reactive Oxygen Species
ROS are highly reactive and are produced even at basal conditions in living organisms by a number of ways. The superoxide anion radical O2− is formed consequent to the one-electron reduction of oxygen. The two-electron reduction product of oxygen in the fully protonated form is hydrogen peroxide (H2O2), and the hydroxyl radical (OH.) results from the three-electron reduction of oxygen.2,3,8,9
Oxygen is reduced to the more reactive superoxide radical by a variety of enzymic and nonenzymic reactions.14 The divalent reduction of oxygen by the enzymes urate oxidase, D-amino-acid oxidase, and glycolate oxidase leads to formation of superoxide. The univalent reduction of oxygen to superoxide followed by the action of superoxide dismutase leads to the formation of hydrogen peroxide. Hydrogen peroxide, though not a free radical itself, can lead to the formation of the more dangerous hydroxyl radical through the Fenton reaction. Autoxidation of dehydrogenases, catechols, thiols, flavins, and oxidases, as well as UV radiation, can also generate superoxide anion and hydrogen peroxide.3,14
Superoxide dismutases are virtually ubiquitous among living organisms. However, there are three SOD metalloisoenzymes, and these isoenzymes display different intracellular and species distributions. Copper-zinc–containing SOD (SOD1) is found in the cytoplasm of virtually all eukaryotic cells. Manganese-containing SOD (SOD2) is located in the mitochondrial matrix of all aerobes. Mammals have extracellular copper-zinc (Cu-Zn) SOD (SOD3) in extracellular fluids or associated with membrane.15 Bacteria possess an iron SOD (FeSOD), a manganese SOD (MnSOD), or both in the cytosol. In addition, higher plants generally contain a Cu-Zn SOD isozyme in the chloroplast. Some plants also have chloroplast FeSOD, chloroplast MnSOD, and leaf peroxisomal MnSOD.15
Anaerobes generally do not have the Cu-Zn SOD or catalase genes. This lack of such genes is shown by their absence from the complete genome sequences now available for the anaerobic Methanococcus jannaschii, Archaeoglobus fulgidus, Pyrococcus horikoshii, Pyrococcus abyssi, and Thermotoga maritima as well as the incomplete genome of Clostridium acetobutylicum.12 However, Photobacterium leiognathi, Caulobacter crescentus,15 as well as the opportunistic pathogen Bacteroides fragilis (the most aerotolerant species among anaerobic bacteria), do have Cu-Zn SOD in addition to Fe SOD.12
Interaction of Reactive Oxygen Species with Lipids
Compromise of the activity of SOD, catalase, or peroxidases by stress or any other factor could result in the triggering of a potentially dangerous pathway of lipoperoxidative damage. Lipid peroxidation has been defined as oxidative degeneration of polyunsaturated fatty acids, set into motion by free radicals. Oxidative damage brought about by ROS is involved in the pathogenesis of several diseases.2–10
The unsaturated acyl chains in membrane phospholipids and cholesterol in membranes, among biomolecules, are highly susceptible to pathologic free radical damage for the following reasons16: First, the inherent structure of polyunsaturated fatty acid chains (polyunsaturated acyl chains are normally unconjugated, and the alpha-methylenic carbons between carbons with double bonds have allylic hydrogen that can readily enter into free radical reaction.13,16 Second, the solubility of molecular oxygen is 700% higher within nonpolar than aqueous milieus (the hydrophobic regions of the membrane are generally the most nonpolar regions of the cell). Third, molecular oxygen has unpaired electrons in the outer orbitals. This feature confers upon oxygen certain properties of free radicals, such as magnetic susceptibility (owing to the magnetic moment of an unpaired electron in orbit) and the propensity to initiate free radical chain reactions among susceptible molecules that lack enough neighboring antioxidant molecules.13,16,17
Oxidation of any polyunsaturated fatty acid results in a number of deleterious end products and is a chain reaction involving initiation, propagation, and termination (Figure 16-1A).17 In the initiation phase, a primary reactive radical molecule (x.) containing an unpaired electron interacts with polyunsaturated fatty acid to initiate the peroxidation process. The reactive radical molecule has enough reactivity to abstract a hydrogen atom from a methylene group (CH2
). An unpaired electron is left on the carbon (
CH
), because a hydrogen atom has only one electron. The carbon radical tends to stabilize itself by molecular rearrangement to form a conjugated diene. The carbon-centered fatty acid radicals combine with molecular oxygen in the propagation phase, yielding highly reactive peroxyl radicals that react with other lipid molecules to form hydroperoxides. Peroxyl radicals are capable of producing new fatty acid radicals, resulting in a radical chain reaction. The peroxyl radicals themselves, in this reaction, are converted to stable termination products (lipid hydroperoxides) (see Figure 16-1A). Thus, the lipid peroxidation process can result in a variety of harmful end products. Markers of oxidative damage include conjugated dienes, isoprostanes, 4-hydroxy-2-nonenal (HNE), HNE-modified proteins, malondialdehyde (MDA), MDA-modified proteins, protein-bound acrolein, ROS-modified DNA, and protein carbonylation.3,4,13,17–19
Interaction of Reactive Oxygen Species with Proteins
Enzymes and other proteins, when subjected to lipid peroxidation in aqueous solutions, undergo polymerization, polypeptide chain scission, and chemical changes in individual amino acids. In spite of the fact that all these chemical reactions are important to the sequence of damage that occurs, current interest focuses on the polymerization or cross-linking of proteins. Pure enzymes undergo cross-linking when exposed to lipid peroxidation, resulting in a several-fold increase in molecular weight in comparison with their original molecular weights. Thus, the biological activities of enzymes and other proteins and their precise arrangement in organelles and subcellular membranes can be lost or impaired by this process. Methionine, histidine, cystine, and lysine are among the most labile amino acids in a variety of proteins.3,17,19
Aldehydic lipid peroxidation products (α,β-unsaturated aldehydes), chiefly the 4-hydroxy-2-alkenals, form adducts with the free amino groups of lysine and other amino acids. Aldehyde-modified proteins are highly immunogenic.3,20,21
Among the 4-hydroxy-2-alkenals the most studied molecule is HNE. This molecule, and related compounds, possesses two very reactive electrophilic sites: the aldehyde group and the alkene bond. The free aldehyde in the open-chain form of the alkenal adduct can react with a second lysine, histidine, or cysteine and then can act as a heterobifunctional cross-linking reagent. The alkene bond reacts via Michael-type addition with the three nucleophilic amino acids cysteine, histidine, and lysine. HNE also reacts avidly with certain antioxidants and enzyme cofactors, including glutathione and lipoic acid (the cofactor for α-ketoglutarate dehydrogenase).3,20,21
Reactive Nitrogen Species
RNS are free radicals that possess biological activity in vivo and are capable of carrying out targeted modification of proteins and lipids. RNS include all nitrogen-based reactive species, such as nitric oxide (.NO) and its nitrogen oxide derivatives, including higher-nitrogen oxide (N2O3) and peroxynitrite (ONOO−).22
The most studied RNS, NO, is a membrane-soluble free radical that is synthesized by nitric oxide synthase (NOS). NOS utilizes oxygen and L-arginine as substrates and converts them to NO and L-citrulline (Figure 16-1B). There are three isoforms of NOS, each transcribed by separate genes. Endothelial NOS (eNOS) and neuronal NOS (nNOS) are the two constitutively expressed isoforms, and inducible NOS (iNOS) is transcriptionally regulated.22–24
NO possesses an apparently contradictory ability—that is, the capacity to bring about both physiologic and pathologic effects. NO effects in vivo largely depend on its concentration and whether it is produced in proximity to other free radicals like superoxide. NO has a direct effect on processes such as proliferation and cell survival at lower concentrations. At higher concentrations, NO has an indirect effect through oxidative stress, leading to nitrosative modification of both proteins and lipids.22–24
Nitrosative modifications refers to selective processes that target precise molecular sites in lipids or proteins for loss or gain of function, in a manner somewhat similar to the well-known phosphorylation or acetylation signal transduction mechanisms. These modifications manifest in proteins, with the exception of heme iron binding, either as S-nitros(yl)ation—the general attachment of NO to nucleophilic centers is defined as nitrosation, and the covalent attachment of the diatomic NO group to reactive thiol sulfhydryl residues in proteins in a redox-dependent fashion is referred to as S-nitrosylation—of cysteine thiols or as nitration of tyrosine residues. Tyrosine nitration occurs through the covalent addition of a triatomic nitro group (NO2) to the phenolic ring of tyrosine residues. Interaction of proteins with NO or other reactive nitrogen intermediates may lead to both S-nitrosylation and tyrosine nitration. N2O3, resulting from reaction of NO with O2, is thought to be a major S-nitrosylating species.22–25
Peroxynitrite, derived from the reaction of NO with superoxide anion (see Figure 16-1B), is also regarded as a major cellular nitrating agent. Myeloperoxidase-catalyzed nitrosonium cation (.NO2), formed from the reaction of nitrite (NO2−) with hydrogen peroxide (H2O2), and nitroso-peroxocarbonate (ONOOCO2−) produced through the reaction of carbon dioxide (CO2) with peroxynitrite are some of the other notable nitrating agents. Data show that lipid peroxyl radicals (LOO.) promote tyrosine nitration by inducing tyrosine oxidation and also by reacting with NO2− to produce .NO2.22–28
Oxidation and Immune Response
Oxidative processes enhance the reaction of the adaptive response. Oxidation of carbohydrates has been shown to the antibody response to co-administered co-antigens. Moreover, the use of the Schiff base-forming agent tucaresol during immunization with protein antigen increases T cell–dependent immune response. Direct modification of protein antigen has been shown to enhance the immune response.3,29,30
Oxidative Damage and Oxidative Modification of Proteins in Autoimmune Disease
Autoimmunity results from the abrogation of self-tolerance and is involved in several human diseases. Autoimmune diseases fall into two categories, organ-specific and systemic. Organ-specific disorders include type 1 diabetes mellitus, thyroiditis, myasthenia gravis, primary biliary cirrhosis, and Goodpasture syndrome, to name only a few. Systemic diseases include rheumatoid arthritis, progressive systemic sclerosis, and SLE. Nearly all these diseases have autoantibodies. Autoantibodies are typically present several years prior to diagnosis of SLE and serve as markers for future disease. Inflammation, infection, drugs, and environmental factors induce formation of neoantigens with involvement of ROS. Thus, oxidative damage is involved in several autoimmune disorders, including SLE.3,4,10,31,32
Free Radical Damage in SLE
The disruption of the homeostasis of reactive intermediates (ROS and RNS) in SLE may lead to a loss of self-tolerance, greater tissue damage, and altered enzyme functions.23
ROS-mediated oxidative damage occurs in SLE.3–10 The greater oxidative damage observed in SLE is mediated by free radicals and is the direct result of a change in the delicate balance between the oxidants and antioxidants and an imbalance in the pro- and anti-inflammatory molecules (Figure 16-2). Oxidative damage in SLE is reviewed in the following sections to show how aberrant generation of superoxide and hydrogen peroxide aided by decreased levels of antioxidant enzymes and antioxidants can lead to the increased production of lipid peroxides (see Figure 16-2), altered fatty acid metabolism, and free radical–induced anti-DNA antibodies.
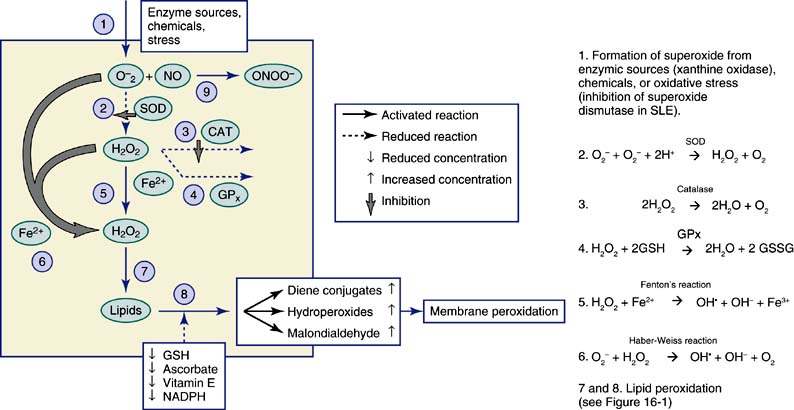
Figure 16-2 Mechanism of free radical mediated oxidative damage. SOD, superoxide dismutase; CAT, catalase; GPx, glutathione peroxidase; O2−, superoxide; H2O2, hydrogen peroxide; NO, nitric oxide; ONOO−, peroxynitrite; GSH, reduced glutathione; GSSG, oxidized glutathione; NADPH, Nicotinamide adenine dinucleotide phosphate (reduced form). Steps 2 to 8 describe the reactions given in Figure 16-2.
Increased Oxygen Free Radical Production in SLE
Normal cellular metabolism produces ROS, especially in the physiologic role of defense against infectious agents and to maintain cellular redox homeostasis.33 Overproduction of ROS, however, results in oxidative stress, which can lead to oxidatively modified neo-autoantigens that can promote loss of tolerance to self and induction of autoantibodies in a variety of diseases, including SLE (Table 16-1).3
In one study, neutrophils of patients with SLE who had clinical manifestations associated with autoantibodies (leukopenia, thrombocytopenia, and hemolytic anemia) were found to have decreased superoxide anion production mediated by the immunoglobulin (Ig) G receptor (Fc gamma receptor [FcγR]) with the cooperation of complement receptors. Neutrophils from patients with SLE who have manifestations associated with the immune complex (nephritis, arthritis, skin symptoms, serositis, and neuropsychiatric disorders), excluding cytopenia, were also found to behave similarly. However, neutrophils from patients with SLE sharing clinical manifestations related to autoantibodies and immune complexes were found to produce significantly more superoxide anion than those from controls. The study concluded that differences in oxidative metabolism of neutrophils brought about by FcγR/complement receptors may reflect an acquired characteristic of SLE linked to specific clinical manifestations.34
Suryaprabha, investigating free radical production, lipid peroxidation, and the levels of essential fatty acids and their metabolites in SLE, found increased levels of superoxide and hydrogen peroxide production by peripheral leukocytes in patients with SLE without any elevations of MDA (as measured by the thiobarbituric acid assay). Furthermore, fatty acid analysis of human plasma showed that both linoleic acid (omega-6 series) and alpha-linolenic acid (omega-3 series) metabolites were significantly lower in the plasma phospholipid fraction of patients with SLE than in controls, suggesting altered essential fatty acid metabolism in these patients.34a,35
Casellas showed in vitro production of superoxide was enhanced in normal and lupus polymorphonuclear neutrophils stimulated with lupus serum. When stimulated by N-formyl-methionyl-leucyl-phenylalanine, lupus polymorphonuclear neutrophils exhibited a 5.2-fold increase in superoxide production over the response of normal polymorphonuclear neutrophils so stimulated.36 The results of this study demonstrate the existence of serum factors in patients with SLE that stimulate O2− production by polymorphonuclear neutrophils. Casellas proposes that increased superoxide production by polymorphonuclear neutrophils in patients with SLE could be important in the development of vasculitis and tissue damage in the disease.36
In addition, several studies show that patients with SLE exhibit increased superoxide and hydrogen peroxide contents in peripheral leukocytes (Table 16-1).8,35 Higher production of ROS can also contribute to oxidative modification of DNA, which becomes more immunogenic and induces antibody production directed against native DNA.37 Studies by Jiang and Chen suggest that excessive free radical production is responsible for the higher lipid peroxide levels in patients with SLE (especially in the active phase of disease) than in controls.10 Gallelli showed significantly higher levels of ROS in patients with lupus nephritis who had carotid plaques than in those nephritis patients without plaques.38 Yet another study observed an increase in superoxide generation during the active phase of SLE with a concomitant decrease in NO levels.4
Altered Antioxidant Enzyme and Antioxidant Levels in SLE
Several studies of SLE have found significantly reduced activities of the antioxidant enzymes SOD, catalase, and glutathione peroxidase as well as reduced levels of antioxidants such as reduced glutathione (Table 16-2), including the study of Shah, who examined North Indian patients with SLE compared to controls.39 Turi found that red blood cell (RBC) SOD and catalase activities as well as activity of reduced glutathione were significantly decreased in pediatric patients with lupus nephropathy.8 A decrease in reduced glutathione and glutathione peroxidase activities was reported in 66 patients with SLE and systemic vasculitides treated with glucocorticoids.6
TABLE 16-2 Antioxidant Enzymes, Inducible Nitric Oxide Synthase (iNOS) and Lipid Peroxidation in SLE
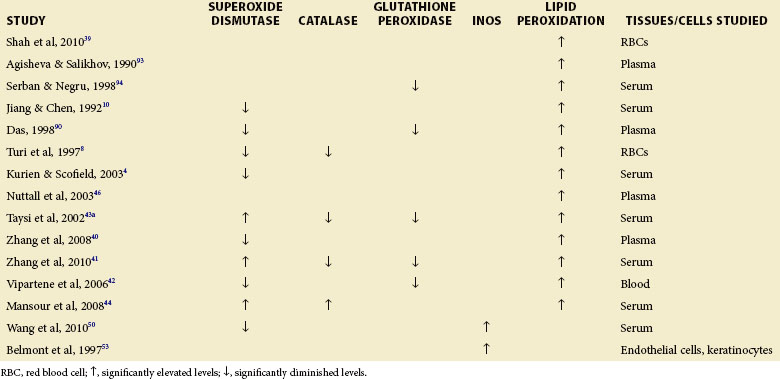
Disease activity has been correlated with antioxidant enzymes and their metabolites. Zhang found significantly lower protein thiol and SOD levels in patients with SLE who tested positive for anti–double-stranded DNA (anti-dsDNA) antibodies than in patients with SLE who tested negative.40 Anti-dsDNA is a frequent correlate of active disease. Others have studied active disease directly. Zhang showed that the disease activity index correlated negatively with superoxide dismutase, glutathione peroxidase, and catalase activities in patients with SLE.41 Vipartene reported that decreased SOD and glutathione peroxidase in patients with SLE may promote oxidative stress.42 When the activities of SOD and glutathione peroxidase were studied during the active and inactive phases of the disease, activity of each was found to be decreased during active disease.35 Agisheva and Salikhov have studied the antioxidant system in 30 patients with SLE by measuring the concentration of alpha-tocopherol and free fatty acids. Shifts in the levels of these antioxidants and lipid peroxidation correlated with the clinical appearance of SLE.35 Kurien and Scofield4 as well as Jiang and Chen10 have also shown decreased SOD1 activity in SLE.
However, some studies have found increased SOD and catalase levels in SLE, suggesting that this protective mechanism could be an adaptation to the greater oxidative stress seen in human SLE. Higher glomerular SOD has been observed in human lupus nephritis, especially diffuse proliferative lupus nephritis.43 Similarly, Taysi showed higher superoxide dismutase activity in the serum of patients with SLE compared to healthy controls.43a Interestingly, in this study, disease activity index in the patients correlated negatively with serum SOD, suggesting that superoxide dismutase is protective. Catalase and SOD activities were also found to be significantly elevated in the sera of patients with SLE in another study.44 Significantly higher glutathione peroxidase activity has been reported in a group of patients with SLE who tested positive for anticardiolipin and in patients with SLE whose Systemic Lupus Erythematosus Disease Activity Index (SLEDAI) scores were higher than 3, compared to patients with SLEDAI less than 3.45
Antibodies to Catalase, Superoxide Dismutase, and Oxidized Low-Density Lipoprotein
Kurien and Scofield4 as well as Mansour 44 have demonstrated antibodies to SOD1 in the sera of patients with SLE. Mansour’s group have, in addition, shown that antibodies to catalase are present in patients with SLE.44 These investigators also found a positive correlation between anticatalase antibodies and antisuperoxide antibodies in patients with SLE. Significantly higher titers of autoantibodies against ox-LDL were found in a group of patients with SLE who tested positive for antibodies against cardiolipin and in patients with an SLEDAI score higher than 3 (Table 16-3).45
Lipid Peroxidation
Numerous studies have found increased lipid peroxidation in patients with SLE. Shah showed a significant increase in the level of lipid peroxidation, measured as MDA, in the erythrocyte hemolysate of patients with SLE.39 The level of MDA correlated positively with SLEDAI score.35,39 Several other studies demonstrated increased lipid peroxidation in erythrocytes and blood plasma of patients with SLE.35,36 Mansour44 reported that MDA and conjugated dienes were significantly higher in the sera of patients with SLE than in healthy controls. Patients with SLE who tested positive for anticardiolipin antibodies as well as patients with an SLEDAI score higher than 3 also had significantly higher thiobarbituric acid–reactive substance levels than healthy controls.44
In a study of oxidative stress in pediatric patients with lupus nephropathy, Turi found an increase in lipid peroxidation in the peripheral RBCs and also a correlation between the presence of active glomerular disease and evidence of oxidative changes in the various parameters measured in peripheral RBCs.8 Serban also found increased lipid peroxidation in patients with SLE who had systemic vasculitides that were treated with glucocorticoid, an increase caused by the dyslipidemias induced by the use of glucocorticoid.6 MDA levels and conjugate dienes have been found to be elevated in patients with SLE.4,10,35
Isoprostanes, products of lipid peroxidation, are thought to be particularly important in the context of vascular disease, and the measure of this parameter has been projected as a reliable, sensitive, and noninvasive marker of oxidative stress in vivo as well as having relevance to vascular disease.35 8-Isoprostaglandin F2alpha (8-iso-PGF2α) has been measured in the serum of 60 patients with SLE and 20 age- and sex-matched controls. The serum concentration of 8-iso-PGF2α was found to be significantly higher in the patients with SLE than in controls. Lipid peroxides were also found to be increased in patients with SLE. Peroxidation of the LDL subfraction may contribute to severe and premature cardiovascular diseases in patients with SLE.46
Protein Modification and Antibodies against Modified Proteins
Specific immunohistochemical studies have clearly shown accumulation of HNE-modified proteins in the dermis of patients with SLE. The observation of intracellular accumulation of HNE-specific epitopes in human SLE for the first time raised the possibility that the higher lipid peroxidation may be directly involved in the pathogenesis of autoimmune disorders.47 Significantly higher HNE-modified protein levels occur in children with SLE.7
Protein-bound carbonyls were found to be elevated in SLE and to correlate with disease activity. Oxidation of proteins is thought to play a role in the pathogenesis of chronic organ damage in SLE.40 For example, MDA, an end product of lipid peroxidation (see Figure 16-2), can be covalently linked to proteins generating intramolecular and intermolecular adducts.48 Elevations of MDA-modified proteins and a significant decrease in the concentration of thiol groups in the sera were observed among 65 patients with SLE (P < 0.05) in comparison with levels found in 60 healthy controls. In addition, the patients with SLE exhibited significantly enhanced levels of IgG antibodies against catalase and SOD-modified proteins (MDA-modified SOD and catalase). Such antibodies are potentially responsible for the increased oxidative damage seen in SLE.49
An analysis of 72 sera from patients with SLE showed significantly higher levels of both anti-MDA/anti-HNE protein adduct antibodies and MDA/HNE protein adducts than sera from 36 age- and sex-matched healthy controls. Interestingly, a higher number of patients were positive for anti-MDA as well as anti-HNE antibodies, and the levels of both of these antibodies were statistically significantly higher among patients with SLE with SLEDAI scores of 6 or more than among patients with SLEDAI scores lower than 6. This study also found a significant correlation between the levels of anti-MDA or anti-HNE antibodies with SLEDAI score (r = 0.734 and r = 0.647, respectively). This observation suggested a possible causal relationship between these antibodies and SLE.50
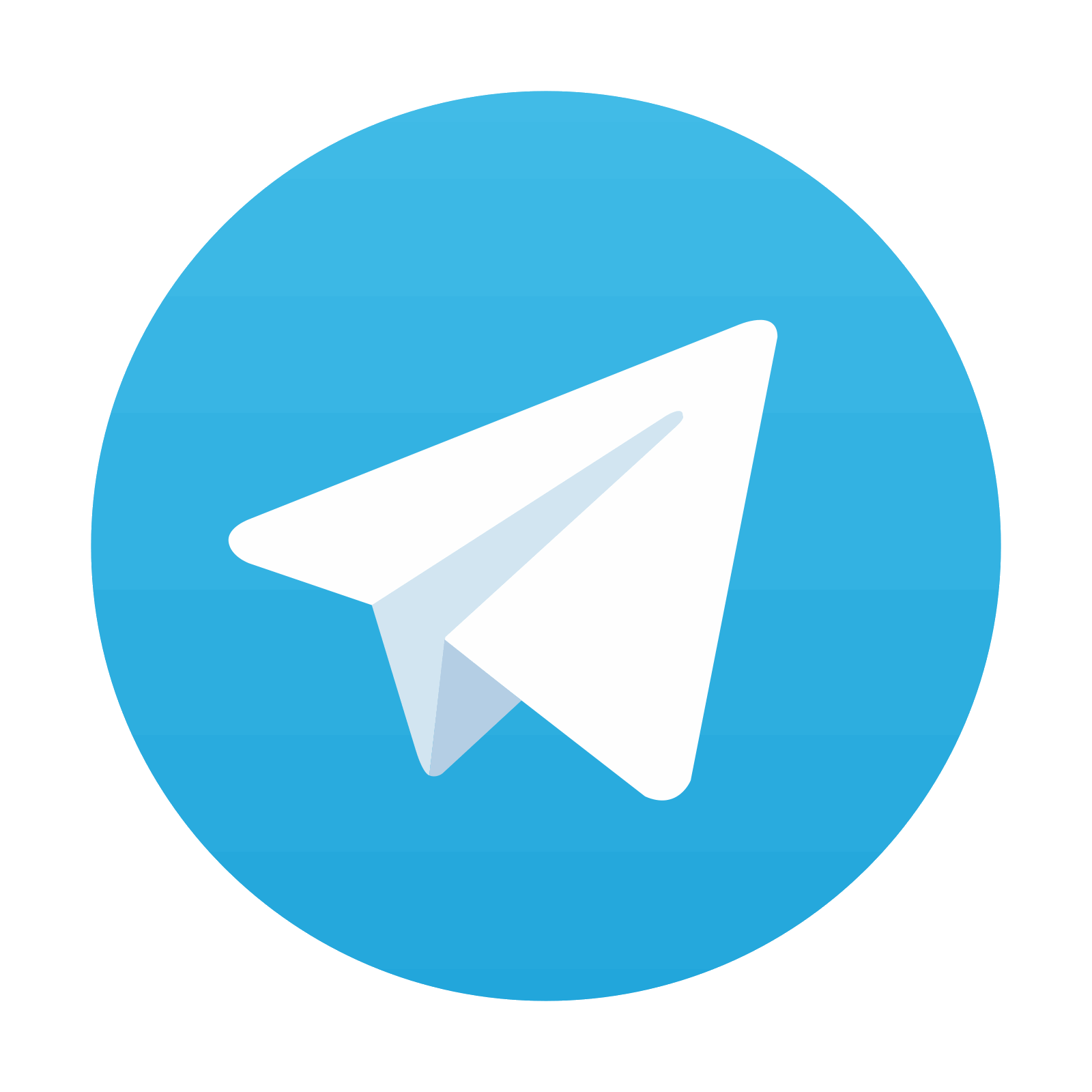
Stay updated, free articles. Join our Telegram channel
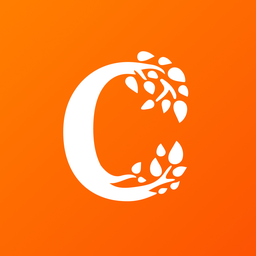
Full access? Get Clinical Tree
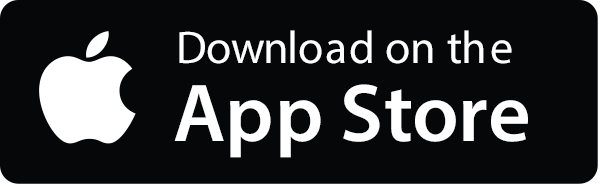
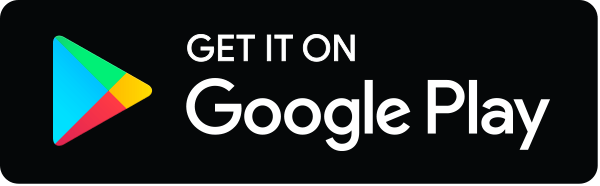