Materials in Hip Surgery
Polymethylmethacrylate
Thierry Scheerlinck
Key Points
Introduction
Polymethylmethacrylate (PMMA) is a methylmethacrylate polymer, better known outside orthopedics by its trade name Plexiglas or Perspex. PMMA has numerous industrial applications, is easy to manufacture, can be made transparent, and has attractive chemical and mechanical properties. In orthopedic surgery and more particularly in hip surgery, PMMA is widely used to fix femoral and acetabular hip implants to bone,1 to fill bone defects,2 to improve the hold of fracture fixation devices in poor quality bone,2,3 and to deliver high local doses of antibiotics4–7 or antitumoral drugs.8
In orthopedic procedures, polymethylmethacrylate or “bone cement” is prepared intraoperatively by mixing a powder and a liquid. The powder (40.0-49.7 g/package9) contains methylmethacrylate polymers and/or copolymers, an initiator, and often additives (dye, radiopacifier, antibiotics).1,9-11 The liquid (14.1-20.8 mL/package9) contains methylmethacrylate monomers, an activator, often a stabilizer, and sometimes a dye1,9-11 (Table 4-1). By combining the initiator in the powder and the activator in the liquid, free radicals are generated and the polymerization reaction is initiated (Fig. 4-1A). During polymerization, the reaction is sustained by the formation of new free radicals from methylmethacrylate (MMA) molecules. This allows the PMMA chains to grow rapidly and to combine. The polymerization reaction ends when the free radicals get depleted by mutual recombination1,9 (Fig. 4-1B). During the polymerization process, the viscosity of the cement increases progressively and limits the mobility of the MMA monomers. As such, just after curing, the polymerized cement still contains 2% to 6% of residual MMA monomer. The monomer may elute from the polymerized mass and be eliminated into the bloodstream, or it may continue to polymerize slowly over the subsequent 2 to 4 weeks.9,12
Figure 4-1 A, When the initiator (BPO) within the powder is mixed with the activator (mostly DMPT) in the liquid, benzoate radicals (R•) containing “unpaired electrons” are created. These radicals start the polymerization reaction by breaking up the C=C bond of methylmethacrylate (MMA), generating new radicals within the MMA molecules. The highly reactive “unpaired electrons” of the MMA molecules combine with new MMA molecules to create large (105-106 g/mol or more) polymethylmethacrylate (PMMA) chains. B, When two PMMA chains containing a free radical combine, the overall quantity of free radicals decreases. The polymerization reaction ends when free radicals are depleted.
The use of bone cement for fixation of hip implants varies widely between geographic regions. In Scandinavia, in European Anglo-Saxon countries, and in New Zealand, most stems and cups are fixed with cement (Sweden: stems 88%, cups 89%13; Norway: stems 73%, cups 82%14; United Kingdom: stems 73%, cups 59%15; New Zealand: stems 73%, cups 40%16). This choice is often justified by data from the literature and from hip registries.17 In Southern Europe, North America, and Australia, cement fixation is much less popular (Canada: stems 29%, cups 3%18; Australia: stems 40%, cups 8%19), and a majority of hip replacements are implanted using “cementless” prosthesis designs. In general, cement is used in the “older, less active population with poor bone quality,” whereas cementless implants are used more often in “young and active patients.”17 However, no consensus has been reached on the exact definition of both these populations.
Despite the excellent long-term survival rates of cemented total hip arthroplasty (THA) (>90% at 10 years and >80% at 15 years postoperatively14,20), the use of bone cement in hip arthroplasty is declining.13,14,18-20 Nevertheless, PMMA continues to be widely used in hip surgery and is unlikely to be replaced in the near future.
This chapter describes the different types of bone cement in terms of their thermal and mechanical properties and the effects of cement shrinkage during polymerization. Then, the effects of additives in bone cement (radiopacifiers, antibiotics, pores) are discussed. Finally, phenomena occurring at the cement-bone and cement-stem interfaces are analyzed. Throughout the chapter, experimental and basic science concepts are described, with an overriding emphasis on practical implications for the orthopedic surgeon. This chapter ends with a list of current controversies and future directions.
Cement Polymerization and Types of Bone Cement
Although polymerization of PMMA bone cement is a continuous phenomenon, it can be divided into four phases: mixing, waiting, application, and setting phases9 (Fig. 4-2). In the mixing phase, the liquid and the powder are mixed until a homogeneous putty is formed. In the waiting phase, the viscosity of the putty continuously increases until it no longer sticks to a gloved finger (dry powder-free latex glove). When the cement is no longer sticky, the application phase has been reached. At this stage, cement dough is delivered to the bone and the implants are inserted.1,9 The application phase lasts until the dough cannot be joined back into a homogeneous mass when kneaded. In the setting phase, the cement hardens progressively and finally maintains a fixed shape. In this phase, shrinkage is commonly observed, and the cement reaches its maximum temperature as a result of the exothermic polymerization reaction. In the setting phase, the implant should be held still to avoid cement cracking or separation of the implant from the cement mantle.
Figure 4-2 The temperature-working curve of high- (Palacos R, Heraeus Medical GmbH, Wehrheim, Germany [top]), medium- (Surgical Simplex P, Stryker, Mahwah, NJ [middle]), and low-viscosity cement (CMW 3, DePuy CMW, Blackpool, UK [bottom]). I, Mixing phase. II, Waiting phase. III, Application phase. IV, Setting phase. The green arrow shows the duration of the application phase at an ambient temperature of 19° C. (Data from Heraeus Medical GmbH and from Kühn KD: Bone cements: up-to-date comparison of physical and chemical properties of commercial materials, ed 1, Berlin, 2000, Springer-Verlag, Fig. 97, p 128, and Fig. 51, p 75.9)
The rate of polymerization of acrylic cement depends on both the temperature and the humidity. The higher the temperature of the cement and/or the ambient atmosphere,1,9,21 the faster the rate of polymerization; the lower the humidity, the longer the application phase.9 For this reason, the International Organization for Standardization (ISO) 5833 standard requires that the working properties of cement be measured at 23° C ± 1° C and 50% ± 10% humidity. The setting time of a PMMA formulation is typically measured using a disk-shaped cement specimen (diameter: 60 mm; thickness: 6 mm) and is defined as the time elapsed between the start of the cement mixing and the moment the temperature within the center of the cement mass reaches half of the peak polymerization temperature.9,21 The duration of each polymerization phase, in relation to the ambient temperature, is specific for each formulation and is represented in temperature-working curves (see Fig. 4-2).
Large variations in the working properties of acrylic cements exist between formulations and between batches of the same formulation, depending on environmental factors. This may explain why surgeons often complain of faulty cement handling properties.9,22 In view of this variability, it is important that joint surgeons become familiar with one formulation of acrylic cement and do whatever is necessary to standardize environmental conditions within the operating theater and the room designated for cement storage. These parameters are often neglected by surgeons, even in countries where cementing is common practice.22
The working properties of cement depend on the chemical composition and the proportions of powder and liquid.9 Currently, three types of PMMA bone cement are used in hip surgery: high-, medium-, and low-viscosity cement (see Fig. 4-2):
• High-viscosity cement has a short waiting phase, as it quickly loses its stickiness after cement mixing. The application phase is long, and cement viscosity starts to increase progressively only toward the end of that phase. The setting phase lasts at least 1.5 to 2 minutes. Therefore, high-viscosity cement is easy to mix in a bowl, to knead manually, and to apply with a “finger packing technique” in the shaft or as a “cement ball” in the acetabulum. However, at room temperature, vacuum mixing and delivery with a syringe are difficult. Precooling the cement to lengthen the various polymerization phases can facilitate this process.1
• Medium-viscosity cement has a low viscosity in the waiting phase and therefore can be vacuum-mixed and delivered with a syringe easily. After approximately 3 minutes, the cement loses its stickiness, and in the application phase, it behaves like a high-viscosity cement. Initially, the viscosity remains almost constant, and at the end of the application phase, it starts to increase progressively. The setting phase lasts at least 1.5 to 2.5 minutes. Thus, medium-viscosity cement combines the convenience of low-viscosity cement for vacuum mixing and syringe delivery at room temperature with the user friendliness of a longer and less constraining application phase.1
• Low-viscosity cement has a long waiting phase in which it remains liquid for a long time. Once the application phase sets in, the temperature and viscosity of the cement increase rapidly, and the setting phase lasts only 1 to 2 minutes. Low-viscosity cement is easy to mix and to apply with a syringe. In the liquid phase, the cement can be pressurized into the cancellous bone. However, it produces a less stable stem-cement-bone construct23 and a weaker cement-bone interface24 compared with high-viscosity cement. This can be attributed to back-bleeding from the shaft, which displaces the low-viscosity dough and contaminates the cement mantle.25 Moreover, liquid cement is difficult to contain, and because of the short application phase, correct timing and control of environmental factors are critical.1 This might explain why low-viscosity cement, in combination with a Charnley stem, has a higher revision rate than high-viscosity cement.26 In past years, interest in low-viscosity cement to fix femoral resurfacing implants has increased. The long liquid phase allows pressurizing of large quantities of cement into the reamed femoral head and facilitates seating of the implant when an implant-filling cementing technique is used.27–29
Properties of Polymethylmethacrylate Bone Cement
Heat Generation
Polymerization of MMA monomer is an exothermic reaction that generates 52 kJ (31-71 kJ) (13 kcal [7-17 kcal]) of energy per mole MMA.9,30 This means that, under the conditions specified by the ISO 5833 standard (23 ± 1° C, 50 ± 10% humidity), the temperature within a cement cylinder of 60 mm diameter and 6 mm thickness can exceed 80° C (52-90° C) during polymerization.9,30 In vivo, the implant, the bone, and the circulation dissipate the heat, and most cement mantles measure less than 6 mm in thickness.31,32 Therefore, lower temperatures have been measured (stem: 40° C [29-56° C]; cup: 43° C [38-52° C])33 or calculated at the bone-cement interface (stem: 45-55° C30; cup: 57° C34). Because a temperature of 50° C for 1 minute or 47° C for 5 minutes can cause bone necrosis,35 the acetabular bone is at risk when a cup is cemented,34 and the endomedullary bone is at risk when cement thickness exceeds 5 mm.30 To avoid thermal bone necrosis, a copolymer cement with a lower curing temperature (Boneloc, Polymers Reconstructive A/S, Farum, Denmark) has been developed.36 However, Boneloc cement has poor mechanical properties,36,37 which caused high failure rates during clinical use.36,38 Today, bone cements advertising a low polymerization temperature are still available (Cemex, Tecres, Italy). However, the gain in curing temperature is small,9,39 and no clinical advantage has been demonstrated.39
Because large quantities of cement can be pressurized into the reamed femoral head, thermal bone necrosis could be an important issue in hip resurfacing.40,41 Finite element analysis predicts peak temperatures up to 54° C for 6 mm of cement penetration, but 74° C for a thick cement mantle associated with a 1-cm3 cyst.42 In vitro, temperatures have been reported to reach median values of 45.4° C (41.6-56.5° C) for thick and 37.2° C (26.6-39.3° C) for thin cement mantles,43 but up to 90° C when cysts were simulated.44 In vivo, temperatures of 68° C have been recorded during hip resurfacing, but pressure lavage, intramedullary suction, and cooling of the resurfaced head allowed adequate temperature control.45 Further research should focus on optimizing the cementing technique to avoid cement congestion of the reamed femoral head during resurfacing procedures.
Cement Shrinkage
During polymerization, the volume of PMMA shrinks by 20.6% compared with the initial volume of liquid MMA.46 However, because bone cement contains only a fraction (±) of MMA as a result of the use of prepolymerized powder, the maximal theoretical volumetric shrinkage is 6% to 8%.46 In practice, cement mixed under vacuum will shrink between 4% and 7%.46,47 When hand-mixed, more air gets trapped within the cement, and volumetric shrinkage tends to decrease.46
Shrinkage during polymerization is important because it can be a source of cement porosity. When cement is constrained during polymerization (i.e., when the outer dimensions of the cement cannot be modified), polymerization shrinkage will induce pores within the cement mantle.46 These pores will compensate for the volumetric shrinkage that could not occur by contraction of the external dimensions of the cement. This particular situation occurs in vivo when cement is inserted into the proximal femur at body temperature while the implant is at room temperature. In this case, cement will start to polymerize at the higher temperature interface (i.e., close to the bone) and will proceed toward the implant. Because the outer layer of cement cures first, it constrains the doughy cement located more centrally and creates shrinkage-induced or type I interfacial defects close to the implant.48 These interfacial pores can act as initiators of fatigue cracks during repeated implant loading and can compromise long-term stem fixation.49 This problem can be solved by reversing the polymerization direction by heating the implant50 or by cooling the bone51 prior to cementation. Our preference is to precool the femoral shaft with saline at 4° C during pressure lavage.
Mechanical Properties
The mechanical properties of PMMA bone cement can be divided into static and dynamic properties. Static properties include the behavior and the resistance of the material when subjected to a pure compressive, tensile, or shear load. Dynamic properties describe the fatigue resistance of bone cement when subjected to repeated loading cycles.
The Static Properties of Bone Cement
Strength and Elasticity.
Three types of static loads can be applied to bone cement: compression, tension, and shear. Under any of these loading conditions, the material first will deform and finally will fail. How bone cement deforms and the ultimate load at which the material fails (ultimate strength [US]) depend on the shape of the specimen, the temperature, the loading regime (compression, tension, shear), the strain rate, the cement composition, the mixing procedure and mixing duration, the duration of aging, and the storage conditions (Table 4-2).9,37,52-56 The ultimate strength of bone cement is about twice as great in compression as in tension or shear. This means that hip implants should be designed to load the cement mantle in compression and to avoid tensile and shear forces.57
Table 4-2
Mechanical Properties of Bone Cement Under Static Loading Conditions
AB−, Without antibiotics; AB+, with antibiotics; D732, according to the ASTM D732 testing specifications; DST, according to a double shear test; ETO, sterilized by ethylene oxide gas;
GI, sterilized by γ-irradiation; 3-pt, 3-point bending test; 4-pt, 4-point bending test.
In predefined circumstances, the relation between the applied load (stress) and the deformation of the material (strain) can be expressed in a stress-strain curve55,58 (Fig. 4-3). Under low compressive and tensile loads, bone cement behaves elastically. This means that deformation of a cement specimen is almost proportional to the load applied, and the stress-strain curve is almost linear (see Fig. 4-3). The slope of the tangent to the initial section of the stress-strain curve is called the modulus of elasticity (E) or Young’s modulus.55 The elasticity modulus of bone cement in compression is similar to that of bone cement in tension (see Table 4-2). The strain at fracture point (emax) represents the degree of deformation (elongation or compression) of the material at failure and is much larger for tensile than for compressive loading.
Figure 4-3 Simulated extension stress-strain curve of polymethylmethacrylate (PMMA) bone cement (green curve [temperature: 54.85° C; strain rate: 0.001/sec]). At low strains, PMMA behaves almost as an elastic material (E), and the slope of the tangent to the initial section of the stress-strain curve (red line) is the elastic modulus. At higher strains, PMMA behaves as an anelastic material (AE), and the stress drops as the result of relaxation (blue arrows). Past the yield strain (εy), PMMA undergoes plastic deformation (P). (Data from Stachurski ZH: Strength and deformation of rigid polymers: the stress-strain curve in amorphous PMMA. Polymer 44:6067–6076, 2003, Fig. 5, p 6072.58)
In practice, bone cement samples are often tested in three-point (e.g., ASTM D790, DIN 53435) or four-point (e.g., ISO 5833) bending. These protocols explore the flexural properties of bone cement (i.e., the combination of compression, tensile, and shear properties). For bone cement, the ultimate flexural or bending strength and the flexural or bending modulus depend on the loading pattern (three- or four-point bending), the cement composition, the mixing modalities, and the duration and storage conditions (see Table 4-2).9,52
Creep and Stress Relaxation.
Creep is defined as “the time-dependent and irreversible deformation of a material under continuous static or dynamic loading”; stress relaxation describes “the time-dependent decrease in stress within a material under constant strain.”1,12,55 Both properties are typical of viscoelastic materials. The amount of stress relaxation is related to the degree of cement polymerization and decreases over the first 4 weeks after cement mixing.12
Bone cement behaves as a brittle viscoelastic material. The initial deformation of PMMA is almost elastic (i.e., reversible and proportional to the load applied) (see Fig. 4-3). However, as the load and the time of exposure to load increase, PMMA behaves as an anelastic material. Under these circumstances, constriction points between PMMA molecules break apart, motion between and within PMMA molecules occurs (molecular relaxation), and stress within the material dissipates (stress relaxation).58 At low strain, when minimal deformation has occurred, the situation is reversible. However, higher loads cause irreversible molecular rearrangements, resulting in a permanent plastic deformation and further stress relaxation. Finally, as the proportion of oriented polymer chains increases, the degree of stress relaxation decreases and the material becomes stiffer again, before it finally fails.58
Creep and stress relaxation are thought to be important for cemented collarless polished and tapered femoral hip implants such as the Exeter (Stryker, Mahwah, NJ) or the CPT (Zimmer, Warsaw, Ind) stem. These stems are designed to subside within the cement mantle and act as a loaded taper.1,57,59,60 Because of creep and plastic deformation of the cement under repetitive load, some subsidence can occur without fracture of the cement mantle. Therefore, the stem transforms axial load into compressive and hoop stresses within the cement. These stresses are transferred to the cortical bone that constrains the cement mantle.1,60 Such a constrained construct seems very effective in resisting both static55 and dynamic loading,60,61 emphasizing the need for the surgeon to achieve cement pressurization up to the inner cortex of the femur. As patients are unloading the cement mantle at night, stress relaxation of PMMA could occur during such periods of inactivity. It has been hypothesized that this might reduce stresses within the cement mantle and thereby may decrease the risk of mechanical failure.1,59,60
Dynamic Properties of Bone Cement
When PMMA bone cement is subjected to repeated loading below its ultimate strength, it can fail progressively by fatigue, as cracks are initiated within the material and propagate to adjacent interfaces. This process is important for hip arthroplasty because (1) in vivo, cement is most often subjected to cyclical loading below its ultimate strength; (2) in vivo fatigue failure patterns can be reproduced in vitro by dynamic mechanical testing below ultimate strength62; and (3) fractographic analysis of retrieved bone cement63,64 indicates that fatigue failure of PMMA and fatigue crack propagation are important failure mechanisms.
The number of cycles needed to fracture a specimen depends on the amount of load applied (i.e., the stress within the material), the loading pattern, the cement composition,65 and the cement porosity (and thus the mixing modalities used for cement preparation).66 The fatigue characteristics of PMMA bone cement can be represented in an S-N curve,52,65,67 which expresses the relationship between the magnitude of the cyclical stress (S) and the number of cycles (N) needed to achieve a given probability of failure (P). The higher the stress, the fewer the number of cycles needed to create fatigue failure (Fig. 4-4).
Figure 4-4 Estimated S-N curve of Surgical Simplex P bone cement for tension loading (temperature: 37° C, sinusoidal loading at 10 Hz). The green dot represents the stress needed to fracture 50% of the specimens after one cycle (i.e., the ultimate strength [σu]) with a fracture probability of 0.50. The stress at the lower asymptote of the S-N curve (blue line) is the endurance limit (σe). Stresses below this limit can be applied “indefinitely” without cement fracture. According to the graph (red line), 10,000 tension loading cycles of 21 MPa are needed to fracture 50% of the specimens. (Data from Krause W, Mathis RS, Grimes LW: Fatigue properties of acrylic bone cement: S-N, P-N, and P-S-N data. J Biomed Mater Res 22:221–244, 1988, Fig. 7, p 23.67)
In clinical practice, hip implants generate repeated stresses within the cement mantle, which cause fatigue cracks within the material and stem-cement debonding. Generally, cement cracks set off at the cement-stem interface49,61 proximally,64 within the metaphyseal region, whereas stem-cement debonding starts around the stem tip distally.64 During the patient’s lifetime, accumulated cement damage and stem-cement debonding will progress toward the middle section of the stem and the implant until the component becomes macroscopically loose.64 This failure mechanism can be reproduced in vitro during mechanical testing62 and simulated with finite element analysis (FEA) models.68 Such models allow differentiation between designs of femoral implants displaying different levels of survivorship in clinical practice.68 FEA modeling can also be used to evaluate the impact of stem design68 and implantation technique61 on device performance, and the contribution to fatigue failure of cement porosity within the bulk of the material66,69 and at the cement-stem interface.49 On the acetabular side, a few studies have reported the results of mechanical fatigue testing70 and dynamic FEA modeling70,71 of cemented cups. These studies predict cup loosening at the cement-bone interface in the superior and posterosuperior regions of the acetabulum70 and increased cement damage with a ceramic cup compared with a polyethylene cup.71 This should encourage the clinician to enhance cemented cup fixation in the posterosuperior region of the acetabulum and to avoid cementing very stiff ceramic72 or metal-backed components.73
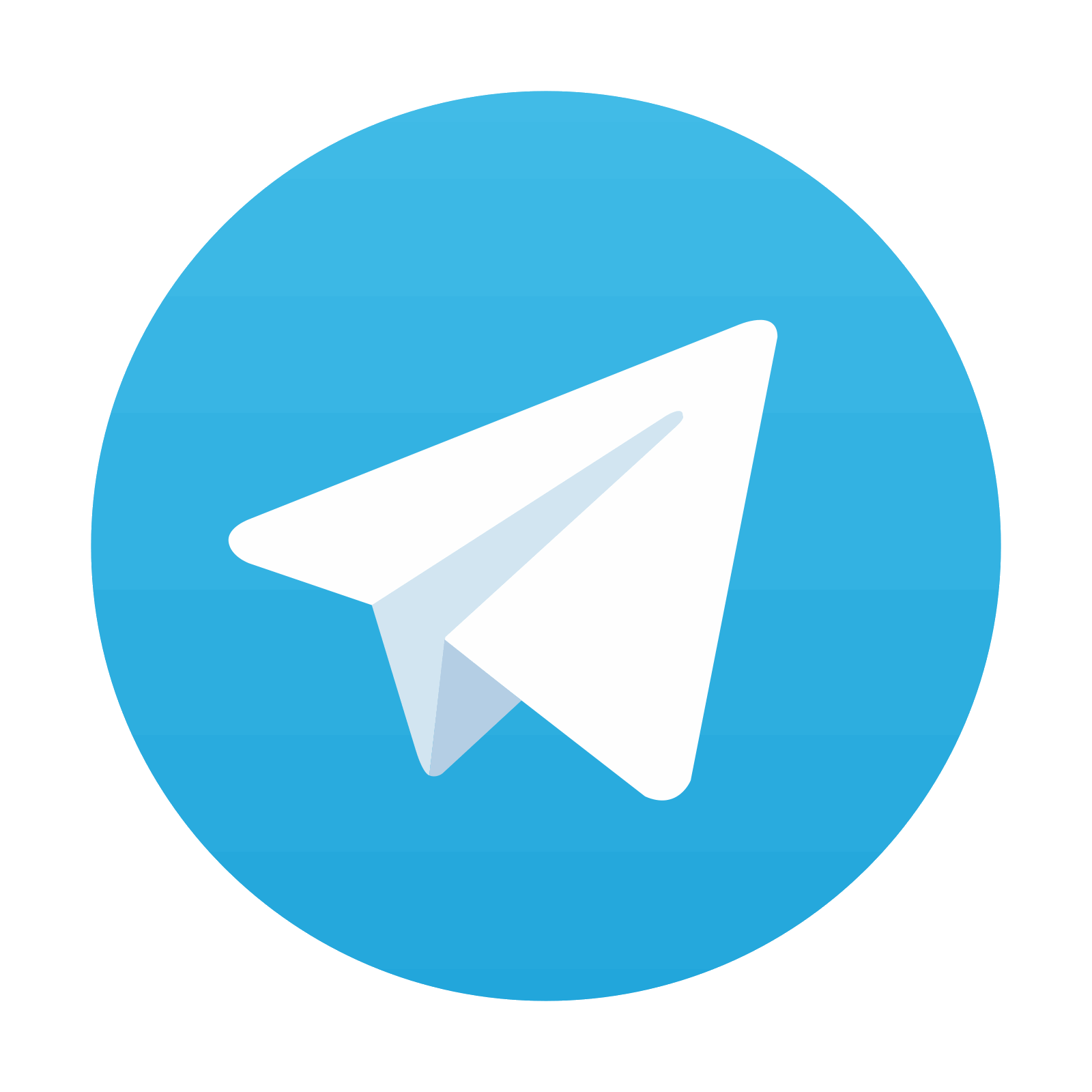
Stay updated, free articles. Join our Telegram channel
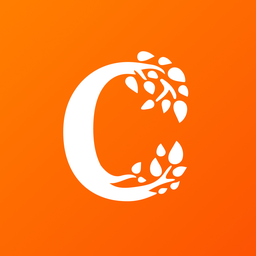
Full access? Get Clinical Tree
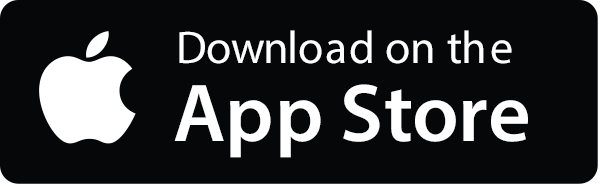
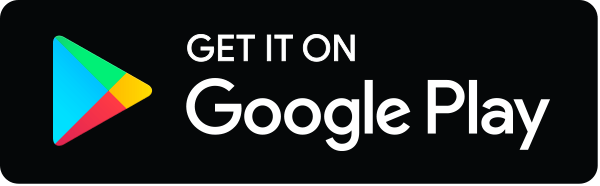