Fig. 11.1
Plots of cortical bone mineral density (Ct.BMD; (a), cortical porosity (Ct.Po; b), cortical area (Ct.Ar; c), and failure load (d) measured at the distal radius with high-resolution peripheral quantitative computed tomography (HR-pQCT) in pre, early, peri, and postpubertal girls and boys. Error bars represent the standard error. (a) p < 0.001; (b) p < 0.01; (c) p < 0.05: significant difference between girls and boys within the same pubertal group. (d) p < 0.001; (e) p < 0.01; significant difference between pubertal group and the prepubertal group within sex. All p values are after Bonferroni correction. Reproduced from Nishiyama et al. [11] with permission from John Wiley and Sons
Rapid changes observed in cortical bone at the distal radius during adolescent growth may lead to a transient period of bone fragility and an elevated risk of fracture [27]. Cross-sectional data from studies that used HR-pQCT suggest that the peak in cortical porosity aligns with the timing of peak forearm fracture incidence in girls and boys [10]. It is thought that despite very high rates of endocortical apposition at the distal radius metaphysis during adolescent growth, bone apposition may be insufficient to increase cortical thickness to a level needed to ensure bone’s strength can withstand mechanical challenges [27]. Thus, it is not surprising that cortical thickness was identified as a risk factor for forearm fractures in children and adolescents [28, 29]. Timing of maturity may also play a role as later menarche was associated with thinner cortices in young adult women [30]. These cross-sectional reports have not been corroborated using prospective data. Thus, longitudinal studies are needed to better characterize changes in cortical bone structure at clinically relevant sites such as the distal radius, and to determine how these changes relate to timing of key maturational events.
At diaphyseal or shaft sites of long bones, resistance to the dominant bending and torsional forces is primarily a function of the distribution and material stiffness of cortical bone [2]. Thus, growth-related gains in bone strength at shaft sites are due, in large part, to increases in bone size resulting from periosteal apposition. This was evident in the early radiographic studies of the second metacarpal by Stanley Garn and colleagues [31, 32]. Periosteal apposition rates (predicted from cross-sectional data) mirrored the trajectory of linear growth, and were greater in boys compared with girls. In a recent 12-year mixed longitudinal study, periosteal apposition (represented by an increase in total bone cross-sectional area by pQCT) at the tibial shaft was greater in boys compared with girls both pre- and post-age at PHV (Fig. 11.2) [16]. Similar findings were also observed over a shorter timeframe across all maturity groups (early, peri, and postpubertal) [15]. Importantly, these data show that boys’ bone size advantage may be apparent as early as prepuberty [12–14, 33]. However, the precise timing of bone strength accrual likely varies by skeletal site [34] and may be influenced by mechanical loads in combination with key hormones such as estrogen, testosterone, and growth hormone [35–37].


Fig. 11.2
A schematic representation of differences in total area (Tt.Ar), medullary area (Me.Ar), cortical area (Ct.Ar), and cortical bone mineral density (Ct.BMD) in boys and girls in relation to maturity offset at −1, 0, 1, and 5 years (from age at peak height velocity). Significant differences between girls and boys are shown for strength-strain index (SSIp), where boys’ values exceeded girls’ at all time points, and Ct.BMD, where girls’ values exceeded boys’ at all time points. (Diagram not exact scale). Reproduced from Gabel et al. [16] with permission from John Wiley and Sons
Notably, the positive exponential relationship between growth in bone width and bone bending strength serves to offset the inverse relationship between bone length and bone strength (Fig. 11.3). Growth in bone length occurs via endochondral ossification at the growth plate and without periosteal expansion slender bones are at an increased risk of fracture [38]. The apparent synchrony between increased bone width (total cross-sectional area) and bone length was illustrated in a 7-year longitudinal study of adolescent girls that used pQCT to assess the tibial shaft [39]. The growth trajectories of bone area and length were similar and both peaked approximately 20 months prior to menarche. This concerted growth may be necessary to avoid potential biomechanical challenges during periods of peak linear growth. Further study is needed to understand the mechanisms underlying this phenomenon and to determine if this relationship is also apparent in boys.


Fig. 11.3
Illustration of the opposite effects of increases in bone width and bone length on bone bending strength. The two rods on the left have the same length, but the larger rod has twice the diameter of the thinner rod; therefore, bone bending strength is eight times greater in the larger rod. The two rods on the right have the same diameter, but a doubling of the rod length decreases bone strength to one eighth of the original value. Reproduced from Rauch [38] with permission
Whereas sex differences in magnitude and duration of periosteal apposition are the driving force behind boys’ bone strength advantage at diaphyseal sites, adaptations on the endosteal surface must also be considered. The early work of Garn and colleagues indicated that girls might experience a greater degree of endosteal bone formation as a result of the pubertal surge in estrogen. A narrower marrow cavity and thicker cortex would in turn provide a calcium reservoir for later reproductive needs. However, some more recent studies that used QCT to revisit these surface-specific changes found something different. Whereas some studies supported Garn’s theory [14, 39, 40], others observed increased area of the marrow cavity, a surrogate for endosteal resorption, in both boys and girls [33, 34, 41]. For example, across 12-years of adolescent growth, medullary area at the tibial shaft (by pQCT) increased in boys and girls both pre- and post-PHV suggesting net endosteal bone resorption in both sexes [16] (Fig. 11.2). It is likely that discrepancies across studies represent a combination of differences in skeletal sites assessed, imaging modalities used, and study design.
Compared with the magnitude of the surface-specific changes that occur during growth, changes in cortical BMD are considerably smaller. In fact, earlier studies that used QCT to assess the mid-femur [42] and the radial shaft [43] reported that cortical BMD remained constant in girls aged 6–15 years. In contrast, results from cross-sectional studies that used pQCT suggest that at the tibial midshaft, cortical BMD is greater in girls compared with boys as early as prepuberty [13] and this sex difference may be more pronounced in the later stages of puberty [14]. Further, more recent evidence from longitudinal studies suggests that gains in cortical BMD (by pQCT at the tibial shaft) occur in both girls [39, 44] and boys [44] during puberty, although to a greater extent in girls (2–4 % over 20-months in girls versus 1 % in peri-pubertal boys). This provides further support for the hypothesis that in the female skeleton, consolidation of cortical bone during puberty provides calcium stores for later reproductive needs. Increased cortical density in girls may also serve to offset the bone strength disadvantage conferred to boys through their larger bone size, on average.
We have learned a great deal from studies that utilized QCT, pQCT, or HR-pQCT to evaluate the growing skeleton. However, the field is relatively young and numerous questions related to the trajectory of bone strength accrual in boys and girls have yet to be answered. An important gap in knowledge relates to the implications of these sex and maturation specific differences for short-term and long-term fracture risk. Well-designed longitudinal studies would serve to clarify the tempo and timing of gains in bone strength at different skeletal sites in boys and girls. In addition, researchers and clinicians must account for the confounding effects of maturation and other key determinants of bone strength acquisition in studies of childhood chronic disease, as discussed below.
What Factors Influence Bone Strength Acquisition During Growth?
Although genetics largely determines our skeletal blueprint [45–49], bone accrual during growth is controlled by functional requirements needed to ensure that bone maintains its mechanical integrity. Conceptually, Rauch and Schoenau’s functional model of bone development [50] is based on Harold Frost’s mechanostat theory [51]. This model purports that increased bone length and muscle force are two major “challenges” that contribute to bone’s integrity during growth. The model suggests that factors such as hormones, nutrition, and physical activity influence bone development through direct effects on the mechanostat strain setpoint or through indirect effects on longitudinal bone growth and muscle force [50]. A comprehensive review of determinants of bone strength accrual is beyond the scope of this chapter and we direct the reader to other excellent reviews of this area [52, 53]. Below, we focus on those factors that have been most frequently assessed in QCT-based studies in recent years.
Muscle
During growth, muscle forces generated during muscular contractions impose the largest loads on the skeleton. This results from muscle continuously working against unfavorable lever arms [51, 54]. In turn, bone must adapt its mass, structure, and ultimately, its strength, to maintain its mechanical competence. The first study that used pQCT to assess bone, noted a strong association between grip strength and distal radius bone area and strength (BSI) in healthy children [55]. Since then numerous cross-sectional [13, 14, 56–60] and prospective studies [15, 39, 61, 62] have investigated the muscle-bone relationship at various skeletal sites using QCT-based imaging. Across these studies, muscle CSA (by pQCT) and lean body mass (excluding BMC, by DXA) were commonly used surrogates of muscle force and were more closely related to bone macrostructure (e.g., total and cortical bone area) and estimates of bone strength, than was BMD.
Should muscle be the primary driver of bone development, gains in muscle force (or a surrogate) during growth would peak prior to the peak for gains in bone strength. This timing differential between muscle and bone was nicely illustrated using cross sectional DXA and hip structure analysis data from the Saskatchewan Pediatric Bone Mineral Accrual Study cohort [63]. However, the only longitudinal study to examine the timing of the muscle-bone relationship (using pQCT) observed something quite different. Growth velocity of muscle CSA (a surrogate of muscle force) peaked 1–8 months earlier than did total BMC and cortical BMD at the tibial shaft. Importantly, this peak was 1 year after the peak in tibial length and total bone CSA in adolescent girls [39]. This suggests a potential differential influence of muscle on specific parameters of bone development. That is, growth in bone size may be less dependent on muscle force whereas bone mineral accrual may be highly dependent on muscle.
There are muscle properties beyond what imaging can assess that likely also play a role. Muscle CSA (by pQCT), as a surrogate of muscle force, may not adequately represent factors such as fiber type and pennation angle that contribute to muscle’s force producing capacity during growth and thus, may not accurately define the loads to which bone is subjected to [58, 64]. In addition, chronic diseases may impact muscle quality, as we describe below. Functional measures of muscle force and power such as those obtained with jumping mechanography or dynamometry should be considered in future studies that aim to assess the muscle-bone relationship.
Nutrition
Intake of total energy as well as specific nutrients such as protein and calcium is essential for optimal musculoskeletal development. These nutritional factors act indirectly on the theoretical mechanostat through their influence on key hormones such as growth hormone (GH) and IGF-1 [53]. However, due to its permissive nature, nutrition likely has the most profound influence on the mechanostat when there is a nutrient deficit. To date, no supplementation trial has investigated the effect of calcium alone on BMD, bone structure, or bone strength. However, there is some evidence to suggest that calcium combined with vitamin D may enhance trabecular BMD at metaphyseal sites in adolescent girls [65, 66].
Similar to calcium, there is insufficient evidence to support beneficial effects of vitamin D supplementation alone on bone structure and strength in the growing skeleton [67]. However, the widespread prevalence of vitamin D deficiency among US children and adolescents [68, 69] may be of particular concern for musculoskeletal health. Vitamin D deficiency may negatively affect bone mass accrual [70] and may also lead to myopathy and subsequent reduced skeletal loading [71]. In the only randomized controlled trial to examine the influence of vitamin D alone, 12-months of supplementation (four doses of 150,000 IU) in postmenarcheal girls did not lead to better bone geometry or strength at distal and shaft sites of the tibia compared with the placebo group, despite improved serum vitamin D levels [71]. We do not yet know whether vitamin D supplementation may benefit bone strength accrual in the less mature skeleton or in children who are vitamin D deficient or insufficient.
Adiposity
We live in an era that has experienced a dramatic rise in childhood overweight and obesity; from 5 % in the 1960s to 32 % in the 1990s [72]. A 33 % higher risk of forearm fracture in overweight children compared with their healthy weight peers has also been documented [73]. Not surprisingly, there is great interest in better understanding the influence of excess fat mass on children’s bone health. However, current studies are conflicting as to whether fat mass benefits or hinders bone accrual. This is likely due to, in part, the reliance on DXA-based studies of BMC and aBMD. Findings are also murky, as these studies did not account for the influence of muscle force when examining the fat-bone relationship.
In the context of mechanostat theory, fat mass represents a static load on the skeleton and as such will not directly influence bone strength [74]. However, overweight/obese children have greater muscle mass for height compared with their normal weight peers, seemingly, to compensate for the increased work needed to move around a greater body mass [75]. In turn, overweight children and adolescents are conferred greater absolute bone strength, adapted to their greater muscle mass, but not to their greater fat mass [62, 74]. This has been highlighted in several cross-sectional QCT-based studies in which positive associations between fat mass and BMD, bone (micro/macro) structure, or bone strength became either nonsignificant [76] or negative [77–79] after adjusting for surrogates of muscle force. Similarly, in a recent pQCT study, bone strength (section modulus) at the tibial shaft was significantly greater in obese (BMI > 97th percentile) adolescents compared with their normal weight peers (BMI < 85th percentile), but when the greater muscle CSA, advanced maturation, and lower levels of physical activity of the obese adolescents were accounted for the bone strength advantage was no longer apparent [80]. The strong relation between muscle and bone is also evident in longitudinal studies: increases in tibial bone strength (by pQCT) during growth were closely tied to change in muscle CSA, and not fat mass, in girls and boys [62]. Thus, in overweight children the question becomes whether in the event of a fall, bones have sufficient strength to withstand the higher load associated with greater fat mass.
While absolute measures of body fat provide an indication of weight status, there is increasing interest in the influence of regional distribution of fat mass on bone health. In particular, fat within skeletal muscle and visceral adipose tissue may be considered pathogenic fat depots with negative implications for bone health. In young girls (9–12 years), higher skeletal muscle fat content (muscle density of the calf and thigh) was inversely associated with 2-year changes in tibial bone strength and BMD (by pQCT) [81]. These associations were independent of other factors that included muscle size, limb length, and maturity. Similarly, visceral adipose tissue was a negative predictor of QCT-derived mid-femur total and cortical bone area and moments of inertia in young women (15–25 years) [82], and abdominal fat (measured with DXA from the pelvis to the base of the skull) was negatively associated with 2-year change in cortical BMD at the tibial shaft in young girls (8–13 years) [83]. Although mechanisms that drive these relationships are unclear, the potentially harmful effects of excess visceral fat on BMD may be mediated by adipokines and pro-inflammatory cytokines [84]. The distribution of body fat changes with advancing maturity and differs between boys and girls [85]. As few studies address this, we perceive a need and an opportunity to further explore these relationships in longitudinal studies.
Physical Activity
Physical activity plays a central role to ensure optimal bone accrual during growth. Evidence to support this is irrefutable and the osteogenic effect of weight-bearing exercise in children and adolescents is summarized in several excellent reviews [86–91]. In particular, pre- and peri-puberty may be the most opportune time for girls and boys to reap the skeletal benefits of physical activity. Exercise-related gains in bone strength in pre- and peri-pubertal children are most often due to adaptations in bone structure (e.g., increases in cortical thickness) rather than bone mass [90]. We know less about bone structural adaptations to exercise in older adolescents, and in light of the well-documented decline in physical activity in this age group [92] future studies that target this population would be invaluable.
Moving forward, researchers might more closely consider the potentially deleterious and independent effects of sedentary behavior on bone strength development. Today’s youth spend as much as 60 % of their waking hours being sedentary while they sit in school, watch TV, or play computer games [93]. The potential consequences of “not loading” the growing human skeleton are not well documented as few studies investigated this relationship [57, 94–96]. Only one study of children that evaluated this relationship used QCT-based imaging [57]. Sedentary time (by self-report questionnaire and accelerometry) was not a significant predictor of tibial bone strength, BMD or macro/micro-structure (by HR-pQCT) in adolescent and young adult males and females. However, levels of physical activity were relatively high in this population. Further study would help to clarify and differentiate between the influence of physical activity versus sedentary behavior on bone, and whether these roles interrelate. For example, is there a threshold for sedentariness above which the consequences to growing bone are more detrimental? We also need to clarify how these complex relationships vary across skeletal sites and levels of maturity.
Is Compromised Bone Strength a Risk Factor for Pediatric Fractures?
As noted in Chap. 1, prevention of pediatric fractures is of great importance particularly given the continued rise in the incidence of distal forearm fractures [97]. Although we currently have insufficient evidence to support the use of QCT-based bone outcomes to predict fracture risk in children [98], deficits in bone strength have been associated with fractures in children in several case-control studies [28, 29, 99]. Compromised bone quality in both the cortical and trabecular compartments may underpin lower bone strength in children with fractures, as smaller bone cross-sectional area [28, 43, 99], lower total and cortical BMD [29, 99, 100], thinner and more porous cortices [28, 29, 101] as well as fewer and thinner trabecule [29, 101] have all been identified as potential risk factors for forearm fracture in otherwise healthy children and adolescents.
Many fractures sustained during growth occur during regular physical activity, but it appears that the etiology of distal forearm fractures during growth differs according to trauma type [28, 29]. Specifically, deficits in cortical and trabecular BMD and structure result in a weaker bone that is unable to sustain loads associated with low- or mild-energy fractures (e.g., a fall from standing height) whereas fractures due to moderate energy trauma (e.g., fall from a bicycle) appear to occur in the context of normal or healthy bone strength (Fig. 11.4). However, recent evidence suggests that in boys, others modifiable factors may also come into play [29]. Poor balance, low levels of physical activity and excess body fat may all contribute to greater forearm fracture risk in young boys [29]. Prospective studies are needed to confirm these relationships and to better characterize the ability of pQCT and HR-pQCT to assess fracture risk during growth. In addition, as young adults with a history of fracture demonstrate lower BMD and bone strength compared with their peers who did not sustain a fracture [102–105], there is a need to determine whether risk factors for forearm fractures in girls and boys track across adolescence and into adulthood.


Fig. 11.4
(a) Bone strength (failure load [N, newtons]) and (b) fall load-to-strength ratio (factor of risk [Φ]) of the distal radius as measured with HR-pQCT in nonfracture controls and boys and girls with a history of either a mild- or moderate-trauma distal forearm fracture. The load-to-strength ratio is calculated as the ratio of the estimated load applied to the outstretched hand during a fall from standing height to failure load derived from finite element analysis of the HR-pQCT scan. Bars represent mean ± SE adjusted for bone age. *p < 0.05; (a) p = 0.075; (b) p = 0.060 compared with the respective nonfracture control group, using Dunnett adjustment for multiple comparisons. Reproduced from Farr et al. [28] with permission from Elsevier
How Do Childhood Chronic Disease and Medications Affect Bone Accrual?
Myriad childhood chronic diseases and their therapies pose threats to musculoskeletal development. The impact may be immediate, resulting in fragility fractures during childhood [106–109], or delayed, due to suboptimal peak bone mass and consequent fractures in adulthood [110, 111]. Improved survival among children with diseases such as malignancies and cystic fibrosis has turned our attention to the prevention and treatment of skeletal comorbidities. Common threats to bone development in children and adolescents across multiple chronic diseases are summarized in Table 4.1. Numerous DXA studies reported abnormal aBMD and BMC in these disorders; however, DXA-based imaging does not provide insight into discrete and site-specific alterations in trabecular and cortical BMD and cortical dimensions that is needed to understand the impact of each risk factor on bone strength and to identify therapeutic targets. To date, the majority of QCT studies in this area focused on children and adolescents with inflammatory disorders who were treated with glucocorticoids, and/or children with muscle deficits. We focus on these key risk factors in this section, and highlight lessons learned from QCT studies.
Glucocorticoids (GCs) are highly effective and widely prescribed for the treatment of numerous childhood diseases; however, they have adverse effects on osteoblast, osteocyte, osteoclast, and muscle cell metabolism. It is well established that administration of GCs results in significant reductions in bone formation due to decreased generation of osteoblasts, impaired osteoblast function, and decreased osteoblast lifespan [112, 113]. GCs also increase osteocyte apoptosis [113]. GC therapy results in an early and transient increase in bone resorption due to enhanced osteoclastogenesis and osteoclast survival, followed by decreased osteoclast differentiation and function [114]. The growing skeleton is uniquely vulnerable to the effects of GCs on bone formation. GC effects on osteocytes and muscle mass may further compromise bone formation through decreased biomechanical loading and impaired signal transduction.
Many prior DXA studies of children treated with GCs were confounded by (a) the negative effects of GCs and the underlying disease on growth, resulting in underestimates of aBMD for age [115], and (b) the impact of the underlying inflammatory disease on bone. Inflammatory cytokines, such as tumor necrosis factor (TNF)-α, have direct adverse effects on osteoblast and muscle cells that are strikingly similar to GC effects [116, 117], with one exception: TNF-α results in prolonged and persistent increases in bone resorption while prolonged GC result in decreased bone resorption [118]. While studies of GC- and inflammation-induced osteoporosis in adults have almost exclusively focused on trabecular bone, studies in children must consider the impact on cortical bone accrual. Based on preclinical data, one would hypothesize that GC therapy and inflammation in children and adolescents would result in decreased trabecular BMD and impaired periosteal expansion as a consequence of decreased bone formation, while inflammation would also result in increased endocortical dimensions due to accelerated endosteal resorption. A series of pQCT studies examined trabecular and cortical BMD, cortical structure, and muscle CSA in children and young adults treated with GCs in childhood for inflammatory diseases, including nephrotic syndrome [119–121], inflammatory bowel disease [115, 122–126], systemic lupus erythematosus [127], and juvenile idiopathic arthritis [128–131]. Table 11.1 summarizes associations between bone and muscle outcomes in these clinical populations.
Table 11.1
QCT studies in children and adolescents with chronic inflammatory diseases treated with glucocorticoids (GC)
Author | Disease (n); measurement site, study design | Trabecular BMD | Cortical BMD | Cortical structure | Muscle CSA |
---|---|---|---|---|---|
Wetzsteon et al. [121] | SSNS (55): tibia pQCT, cross-sectional | Decreased | Increased | Increased periosteal circumference in association with increased muscle and fat area | Greater than reference participants, associated with GC-induced gains in fat CSA |
Tsampalieros et al. [133] | SSNS (56); tibia pQCT, longitudinal | Decreased | Increased; positively associated with GC dose | Greater increases in tibia length were associated with decreases in periosteal circumference Z-scores | Greater than reference participants, associated with GC-induced gains in fat CSA |
Dubner et al. [123] | Crohn’s disease (78); tibia pQCT, longitudinal incident cohort | Decreased at diagnosis, improved partially during the first 6 months of therapy | Normal at diagnosis, increased with GC therapy | Increased endosteal circumference at diagnosis. endosteal circumference improved with therapy but periosteal circumference lagged | Decreased at diagnosis, improved partially with therapy |
Tsampalieros et al. [124] | Crohn’s disease (55); tibia pQCT, longitudinal incident cohort | Persistent deficits at 3–4 years despite little clinical disease activity | Decreased in association with improvements in cortical area | Periosteal circumference comparable to controls at 3–4 years; endosteal circumference remained increased | Persistent deficits at 3–4 years |
Werkstetter et al. [125] | Crohn’s disease and ulcerative colitis (102); radius pQCT, longitudinal mixed incident/prevalent cohort | Decreased | Increased | Preserved cortical area adjusted for height. The lack of adjustment for age potentially resulted in the comparison of pubertal inflammatory bowel disease participants with prepubertal controls, masking cortical deficits | Decreased muscle CSA |
Burnham et al. [129] | Juvenile idiopathic arthritis (JIA, 101): tibia pQCT, cross-sectional | Decreased | Increased | Decreased periosteal circumference in polyarticular JIA and spondyloarthritis | Decreased in polyarticular JIA and spondyloarthritis |
Stagi et al. [127] | Systemic lupus erythematosus (56); radius pQCT, cross-sectional | Decreased; negatively associated with GC dose | Increased; positively associated with GC dose | Decreased cortical bone area; no information on periosteal or endosteal circumference provided. | No different from controls; negatively associated with GC dose |
Persistent inflammation and elevated cytokine levels characterize childhood diseases treated with chronic GC such as inflammatory bowel disease and juvenile idiopathic arthritis. In contrast, steroid sensitive nephrotic syndrome (SSNS ) responds promptly and completely to GC therapy, and the nephrotic state is quiescent during high-dose therapy. Unfortunately, SSNS relapses in the majority of children when the GC dose is reduced, resulting in protracted and repeated courses of therapy. Although relapses are associated with transiently increased cytokines levels, these abnormalities promptly resolve with GC therapy and disease remission [132]. Therefore, SSNS serves as a clinical model, with minimal systemic inflammation, to examine the independent effects of GC on BMD and structure during growth.
In this clinical model, pQCT imaging in children and adolescents with SSNS demonstrated significantly lower trabecular BMD and higher cortical BMD at the distal and shaft sites of the tibia, respectively, compared with healthy children [121]. Further, bone formation and resorption biomarkers were significantly and inversely associated with cortical BMD in SSNS and controls and were significantly lower in the 34 SSNS participants taking GCs at the time of the study compared with controls. The authors attributed the elevated cortical BMD to lower bone formation and greater secondary mineralization [121], a hypothesis that was supported by findings from a longitudinal follow-up study [133]: greater GC dose, lesser increases in tibia length and lesser increases in cortical area were significantly and independently associated with greater increases in cortical BMD in SSNS. Subsequently, other studies confirmed an association between GC therapy and increased cortical BMD in children with other chronic diseases [125, 127, 134]. Of note, posteroanterior spine aBMD did not differ between SSNS participants and controls, likely due to the superimposed lower trabecular BMD in the vertebral body and higher cortical BMD in the cortical shell and spinous processes [121]. These data highlight the utility of pQCT to capture GC effects that are not evident with DXA.
The clinical model of SSNS also provides the opportunity to examine how the muscle-bone relationship is affected by chronic disease and GC therapy. Compared with their healthy peers, children with SSNS had elevated BMI and lower extremity fat CSA (by pQCT), consistent with GC effects. In turn, similar to overweight and obese healthy children, children with SSNS had greater muscle CSA [135], which was associated with greater periosteal circumference at the tibial shaft. However, when these relationships were explored in the follow-up study, gains in tibia length were associated with declines in periosteal circumference Z-scores in children treated with GC for SSNS. These data suggest that the greater muscle mass (secondary to greater fat mass) in children treated with GC for SSNS promoted bone accrual, but GCs prevented normal gains in periosteal circumference during linear growth. Although GCs are known to impair muscle mass and function, a subsequent analysis in these SSNS patients demonstrated normal muscle strength relative to muscle CSA, assessed using ankle dynamometry measures of peak torque relative to pQCT calf muscle CSA [136].
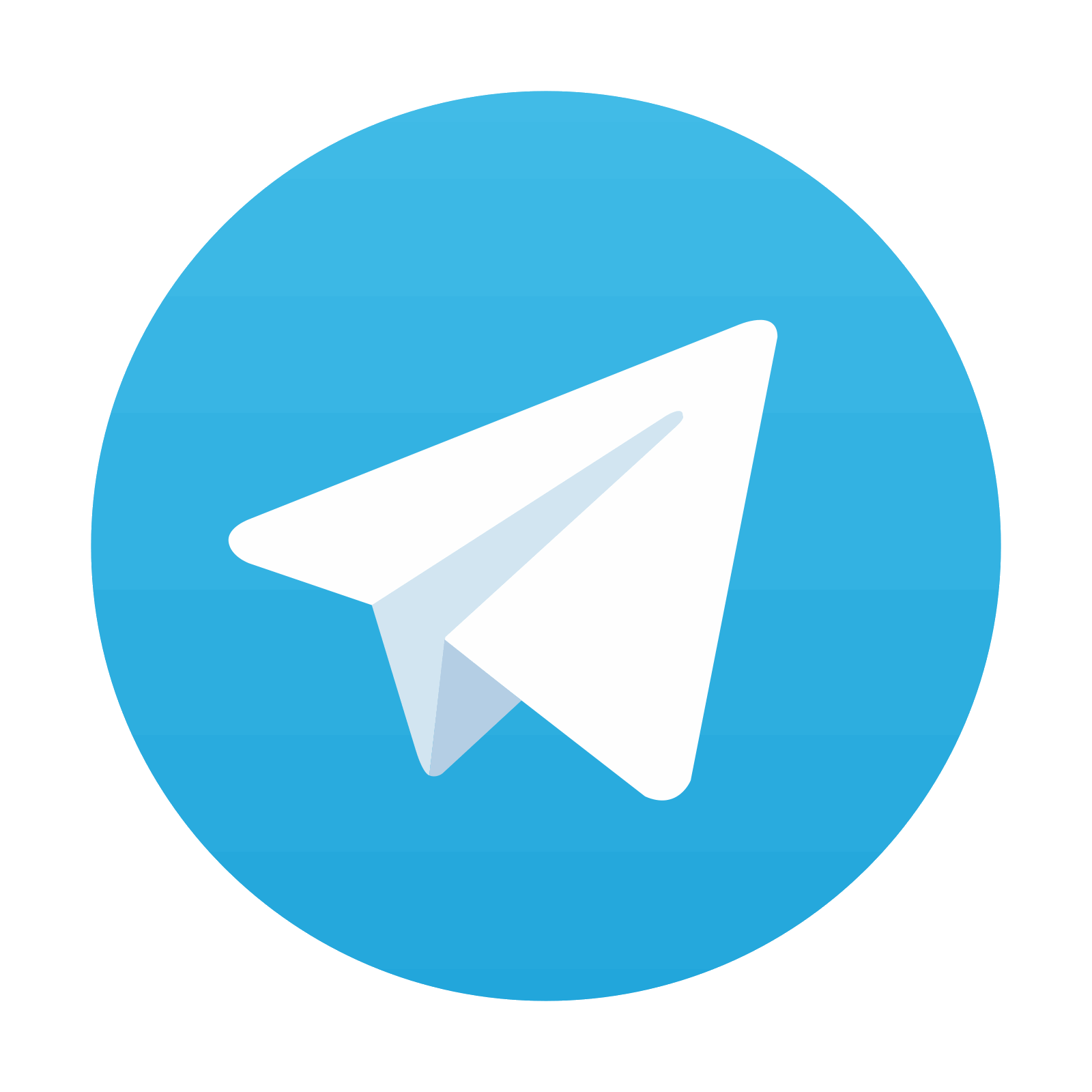
Stay updated, free articles. Join our Telegram channel
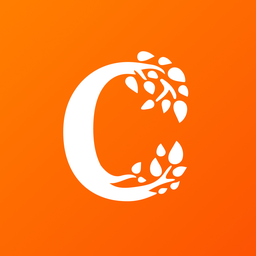
Full access? Get Clinical Tree
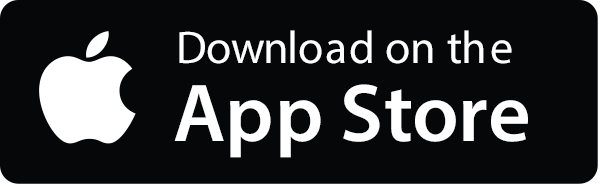
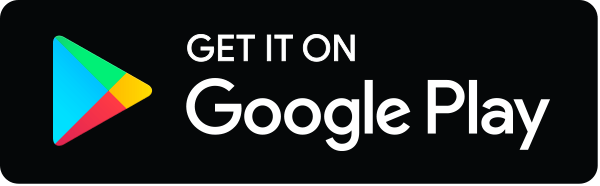