Date range
2011
7
5
2006–2010
26
11
2001–2005
7
3
1996–2000
8
0
1991–1995
6
na
>1990
4
na
22.3 Organ Culture Model Development
Organ culture models have been developed over the last few decades using animal discs of various sizes in either simple or complex bioreactor systems to provide controlled in vitro conditions that simulate the in vivo situation. The earliest organ culture systems faced many challenges in establishing effective and relevant culture conditions that were adequate to maintain tissue integrity and intervertebral disc cell viability and metabolism. The most modern systems are computer controlled and capable of maintaining multiple intervertebral discs from large animals with loading in multiple degrees of freedom. The following sections provide an overview of the historical progression and diversity of organ culture models that have been developed.
22.3.1 Choice of Animal Models
When compared to the human, small animal models (including mice, rats, and rabbits) have enhanced nutrient and waste transport characteristics. Small animal models also have higher matrix water content, greater distinction between annulus fibrosus and nucleus pulposus, and a larger number of notochordal cells that persist to older ages; the latter cells exhibit an elevated metabolic activity when compared with chondrocytic nucleus pulposus cells (Aguiar et al. 1999; Oegema et al. 2000). Indeed, the notochordal population in human intervertebral discs is greatly reduced if not entirely absent by puberty (Hunter et al. 2004). Sakai et al. (2009) found considerable interspecies differences when comparing gene expression levels of notochordal and non-notochordal animal discs with cells of human intervertebral discs. The high metabolic rate, relatively rapid aging process, and low cost of small animal models are all very attractive research features. However, these advantages ignore major differences between animal and human disc cells. From this perspective, therapeutic interventions that may be successful in small animal models may not succeed in large animal models or humans. Depending on the biologic or biomechanical question, small animal models can also be of limited value as they lack sufficient tissue for multiple measurements.
The intervertebral discs of large animal models are of comparable size to the human, and nutrient transport, disc structure, cellularity, and metabolic rates are similar. Nutritional and transport limitations in the large human intervertebral discs have long been considered a key factor in slowing successful repair (Kandel et al. 2008) and in contributing to the uniquely challenging disc cell niche. These large diffusion distances that human disc cells experience are likely to become a critical issue as research becomes more clinically translatable. Recently developed bioreactor systems are designed to handle the size and loading requirements of bovine and ovine intervertebral coccygeal discs which are among the most commonly used tissue sources.
There are differences in the types of loading that intervertebral discs of quadrupeds experience when compared to bipeds. This difference reflects the orientation of the spine with respect to gravity and biomechanical differences between anatomic regions (tails vs. spines). Surprisingly, since the dominant loading forces on the spine of all animals are associated with muscle action along the axis of the spine, these biomechanical differences are often smaller than one might expect and are most relevant when considering instrumentation design. Even loading on the tail is dominated by axial musculature, so that loading differences between ovine, bovine, rodent, and other coccygeal intervertebral discs models are typically no more significant than the biological differences between species. These biomechanical similarities are highlighted by the findings that the effective axial stress (axial force normalized by cross-sectional area) required to restrain swelling of tail intervertebral discs (0.1–0.3 MPa) is similar to the in vivo intradiscal pressure measured in human lumbar discs in the prone position (0.1–0.3 MPa; see also Sect. 22.3.4). The range of motion and peak stresses do vary across intervertebral discs levels and species (Alini et al. 2008; Demers et al. 2004; MacLean et al. 2005; Oshima et al. 1993; Wilke et al. 1999). These differences are paramount when considering final geometry and material choices for instrumentation and implants at final stages of product development.
Most commonly, the intervertebral discs of large animals are smaller in size than human lumbar discs. However, human intervertebral discs have a greater disc height (height = 11.3 ± 0.3 mm) and they are elliptical in cross section (medial-lateral diameter = 55.9 ± 9.4 mm and anterior-posterior diameter = 37.2 ± 4.7 mm). Bovine caudal discs are smaller than human lumbar discs (bovine disc height = 6.90 ± 0.35 mm) and circular in cross section (diameter = 28.9 ± 2.0 mm). The normalized transport distance or aspect ratios (disc height normalized to lateral diameter) are similar for human (0.202) and bovine (0.239; O’Connell et al. 2007). A comparison of intervertebral disc dimensions and differences in tissue composition of commonly used animal models is shown in Fig. 22.1. The most obvious difference between the discs is the size: bovine coccygeal discs have diameter of ∼22 mm (30 % smaller than human discs), followed by lumbar porcine (∼18 mm), coccygeal ovine (∼15 mm), rat (∼4 mm), and mice (∼2 mm). The coccygeal discs of bovine and ovine are approximately round in the transverse plane compared to the human and porcine which are kidney bean shaped and typically approximate to an ellipse. Similar to the healthy human nucleus pulposus, the nucleus of bovine and ovine coccygeal intervertebral discs appears white and fibrous, whereas the gelatinous nucleus of the porcine and rodent lumbar discs clearly differs in composition. Several authors have provided a more comprehensive comparison of bovine coccygeal intervertebral discs with the human (Demers et al. 2004; Oshima et al. 1993). Furthermore, most smaller species retain notochordal cells throughout aging, while bovine and ovine species do not (Hunter et al. 2004).
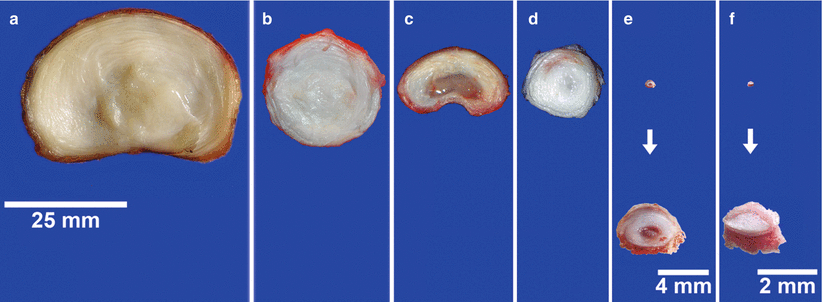
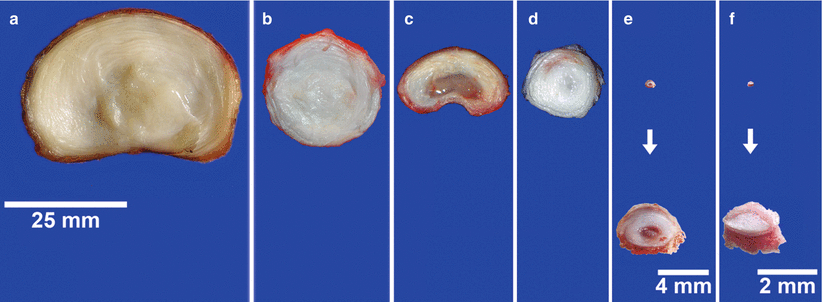
Fig. 22.1
Disc species comparison. Transverse sections from (a), human (lumbar); (b), bovine (coccygeal); (c), porcine (lumbar); (d), ovine (coccygeal); (e), rat (lumbar); and (f), mouse (lumbar) intervertebral discs
Taken together, coccygeal intervertebral discs from bovine and ovine are of large size with similar aspect ratios, transport distances, and composition as human discs and are becoming accepted tissues for large animal organ culture systems.
22.3.2 Intervertebral Disc Swelling
A significant challenge for intervertebral disc research, regardless of species, is to maintain the original tissue structure. When muscular loading is removed and intervertebral discs are isolated, the tissue exhibits a propensity to swell in standard culture media as a result of development of large osmotic gradients (Urban and Maroudas 1981). Notably, if the disc is isolated without the superior and inferior endplates, its weight increases drastically compared to discs with bone-covered endplates. This rapid increase in wet weight of intervertebral discs without endplates is apparent within the first 1.5 h (% increase of initial wet weight for discs after 10, 20, and 90 min was 10, 16, and 22 % for intervertebral discs without endplates, while the change was 2, 4, and 5 % for discs with bone-covered endplates (Fig. 22.2)). After 15 h, the weight of discs without endplates increased around 22 % compared to 10 % when cultured with bone-covered endplates. A similar finding was observed by Gawri et al. (2011) who compared swelling of intervertebral discs with cartilage endplates vs. discs without endplates; after 66 h in culture, the observed increase in wet weight was 21 % in the cartilage endplate group, compared with 44 % for the discs without endplates (Gawri et al. 2011). When disc tissue was isolated as fragments, the wet weight was elevated to an even greater extent (Urban and Maroudas 1981). In response to swelling, disc cells increase expression of catabolic and pro-inflammatory genes and can undergo cell death (Haschtmann et al. 2008a). Conversely, as the disc dehydrates in air, exposure to aqueous media is required to maintain tissue structure and biomechanical integrity (Pflaster et al. 1997). To counter or prevent this propensity for swelling and preserve the physiological structure of the intervertebral disc, ex vivo, several strategies have been developed.
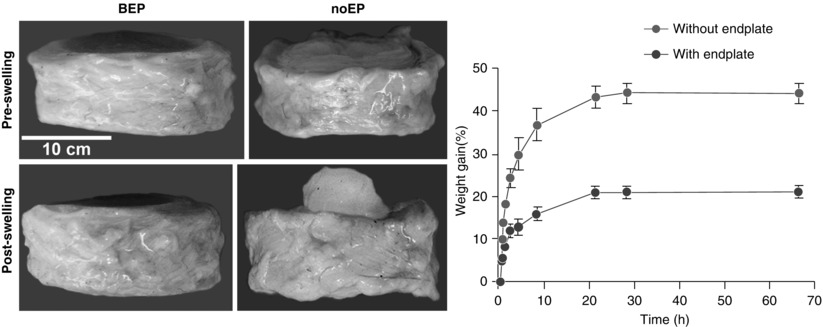
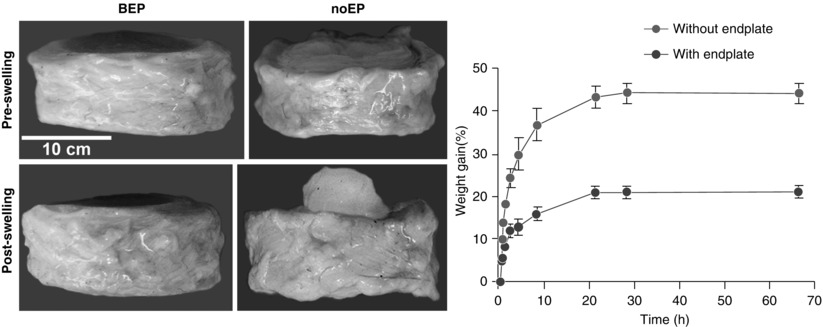
Fig. 22.2
Free swelling of disc explants. Left: comparison of swelling capacity and deformation of intervertebral discs with and without bone endplates. Right: change in wet weight of intervertebral discs without endplates and cartilage endplate cultures after 66 h (n = 4, error bars indicate standard deviation; modified from Gawri et al. 2011)
22.3.3 Free-Swelling and Osmotic Loading Models
To evaluate cell metabolism and protein synthesis, intervertebral discs have been cultured in media as intact organs (Oegema et al. 1979). Despite the swelling which can disrupt structure and promote loss of glycosaminoglycans (GAGs) and structural proteins, cells in the disc remain metabolically active (Chiba et al. 1998). Chiba and coworkers cultured whole rabbit intervertebral disc for up to 1 month in alginate to inhibit swelling using techniques adapted from more traditional cell culture protocols. In this system, tissue integrity and biosynthetic activities were maintained. However, other investigators indicated that this technique, and its general nonphysiological approach to inhibit swelling, required further optimization. A new approach using hyperosmotic media (supplemented with TGFβ) to inhibit swelling was described by Risbud et al. who cultured rat lumbar intervertebral discs for 1 and 3 weeks. After 1 week, no differences in cell viability, morphology, or gene and protein expression profiles were found between cultured and control discs, whereas after 3 weeks in culture, a loss of cell function was observed (Risbud et al. 2003). This was a novel approach for investigating the nucleus pulposus function under physiological and pathophysiological conditions. The model also demonstrated that even if swelling of the intervertebral disc could be prevented, cell viability could not be maintained, suggesting that inhibition of swelling was not sufficient to maintain physiological levels of nucleus pulposus cell viability and function in long-term culture.
To prevent swelling of the intervertebral disc in small animals, a further refinement of this technique involved retention of the surrounding tissues. Lim et al. (2006) cultured rat motion segments (the disc with the adjacent vertebrae) for 4 weeks under free-swelling conditions; they reported that after 14 days, nucleus pulposus and annulus fibrosus cell viability (∼95 %) was maintained; after 21 days, only 25 % of the cells were viable and viability decreased even further after 28 days (9 %). Thus, the authors demonstrated that rigid fixation of the disc through the vertebral bodies inhibited tissue swelling in media. However, similar to the study by Risbud and coworkers, the loss of cell viability was problematic at longer time intervals. Together, these studies support the concept that reduced nutrient and metabolite transport through the vertebrae and annulus in these models is a problem. It is also notable that endplate transport may be a limitation even in these small animal models which have diffusional transport distances much smaller than human and large animal models.
Haschtmann et al. (2006a) cultured rabbit intervertebral discs with retained endplates in standard media under free-swelling conditions. It was hypothesized that the endplate served to “naturally constrain” the nucleus pulposus. These workers observed that cell viability could be maintained for at least 4 weeks without losing structural integrity or matrix composition. However, “degenerative” gene expression pattern and lowered metabolic rate were reported, thus limiting the use of this model for the study of the pathophysiological and long-term changes in the disc (Haschtmann et al. 2006a). In a subsequent study, rabbit intervertebral discs were cultured with endplates with diurnal changes in hyperosmotic conditions. These conditions were better able to maintain aggrecan gene expression and inhibit swelling and collagen I expression than conventional static osmolarity conditions (Haschtmann et al. 2006b). This finding suggests that the amount of loading and the way the load was applied has a large effect on disc biosynthetic activity.
In a further study by Van Dijk et al. (2011), swelling of nucleus pulposus tissue explants was inhibited using culture media made hypertonic by supplementation with polyethylene glycol. Tissue integrity and cell viability of these bovine explants was maintained for 21 days. However, the decrease in matrix gene expression observed in this tissue explant model (a best case scenario for nutrient transport since there were no endplates or annulus fibrosus to impede nutrient transport) highlighted that biomechanical stimulation, in addition to nutrient transport, is important to promote anabolic metabolism of the intervertebral disc.
22.3.4 Static and Diurnal Compression Loading Models
Oshima et al. (1993) evaluated the axial load required to prevent swelling. These workers estimated that the intervertebral disc experiences an effective mechanical stress of ∼0.3 MPa in vivo. In a short 12-h study, both tissue hydration and matrix synthesis were affected by varying the static axial loading. In vivo levels were maintained when the effective stress ranged between 0.13 and 0.26 MPa (Ohshima et al. 1995). This early work established what is now considered to be appropriate baseline loads for large animal disc organ culture and showed the feasibility of this model for studies of the mechanobiology of the intervertebral disc (Fig. 22.3a). Baseline axial compression levels of similar values ∼0.15 MPa were also found to retain in vivo disc height in rat caudal discs (MacLean et al. 2005). From these and other studies, it was concluded that for a number of animal species and vertebrae levels, to prevent disc swelling, an effective compressive stress of ∼0.1–0.2 MPa is a reasonable baseline loading condition.
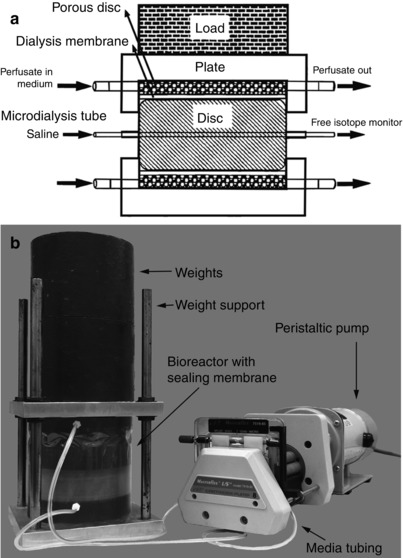
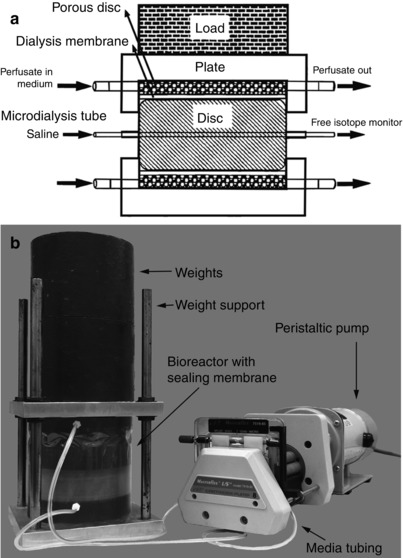
Fig. 22.3
Static and diurnal organ culture models. (a) Schematic representation of the first axial compression bioreactor for large animal organ culture designed by Ohshima et al. (1995). (b) Load frame to allow diurnal loading on the Ohshima-style static bioreactor
Lee et al. (2006) created a bioreactor system for longer-term culture using the same static compression loading design of Ohshima and coworkers. This system successfully maintained cell viability for up to 7 days; although the GAG synthesis rates dropped by 70–80 % after only 2 days of culture, the cells remained sensitive to mechanical stimulation. Lee and coworkers concluded that this drop in GAG synthesis was the result of static loading, since in vivo experiments demonstrated decreased cell metabolism with loading and enhanced biosynthesis under dynamic compression loading (Maclean et al. 2004; MacLean et al. 2003). Lee and coworkers also evaluated the possibility of culturing intervertebral discs with intact endplates, which by anchoring the annulus fibrosus fibers and retaining nucleus pulposus, pressurization would maintain the biomechanical integrity of the disc. However, culturing discs with endplates drastically reduced cell viability within 1 week, especially in the nucleus. The reduced viability was hypothesized to be associated with formation of blood clots in the vertebral endplates that inhibited nutrient transport. A further validation of bovine organ culture explants without endplates was performed by Korecki et al. (2007; Fig. 22.3b) who found that static as well as diurnal loading conditions maintained GAG content and had similar cell metabolism rates.
22.3.5 Improved Dissection Procedures for Disc Organ Culture
As nucleus pulposus viability in organ culture requires the retention of the endplate cartilage, it was necessary to improve current methods for isolating these regions of the intervertebral disc. Gantenbein et al. (2006) obtained intervertebral discs from sheep that had been treated with a systemic anticoagulant before death. The endplates were cleaned thoroughly with a debridement tool to ensure vasculature channel competence and facilitate nutrient transport. Intervertebral discs with endplates were cultured either under static (0.2 MPa/24 h) or diurnal loading (0.2 MPa/6 h and 0.8 MPa/16 h) for 4 days in media supplemented with 50 mol/l of dextran conjugated with a fluorescent label. They demonstrated that when discs are loaded diurnally, the fluorophores diffused to a greater extent through the annulus fibrosus and endplate towards the disc center than when loaded statically. This was the first study to demonstrate a technique in which (1) large animal intervertebral discs could be cultured with endplates under diurnal loading conditions and (2) cell viability and GAG synthesis could be maintained for 7 days. However, gene expression data indicated possible catabolic changes in cell activity, which was likely associated with a lack of cyclic loading stimulus.
Free-swelling studies by Gawri et al. (2011) on human intervertebral discs and Jim et al. (2011) on bovine discs evaluated if the cartilaginous endplates promoted tissue structure and cell viability and metabolism. In both studies, the vertebral endplates were prepared by removing bone with a high-speed drill until only the cartilage endplates remained. Disc explants with cartilage endplates in free-swelling conditions were cultured for up to 4 months in medium containing high or low glucose levels with high or low concentrations of fetal bovine serum (FBS) (high glucose = 4.5 g/l; low glucose = 1 g/l; high FBS = 5 %; low FBS = 1 %). Importantly, cell viability of these free-swelling explants with cartilage endplates was very high (>95 %) for up to 4 months for all nutritional culture conditions, indicating that adequate waste and nutrition exchange was taking place. However, after 4 weeks in culture, the increase in disc height was about 21 %, and matrix degradation was observed to have increased, independent of the various culture conditions. Similar to other studies, they reported high cell viability but GAG loss in free-swelling culture conditions, suggesting that physiological axial loading may be necessary to regulate cellular metabolism and retain GAG content.
22.3.5.1 Dissection Procedures That Retain Structural Integrity of the Explants
The method of removing and preparing discs from the surrounding tissues is an intricate multistep process. The procedure utilizes bovine caudal intervertebral discs; these discs are freely available and similar to the human lumbar disc (Sect. 22.3.1). It can be adapted for use with other animals, other spine levels, and human specimens. As demonstrated by Lee et al. (2006), without thorough cleaning of blood clots within the endplates, there is inhibition of nutrient and waste transport and nucleus pulposus cell death. In contrast to the earlier techniques that serve to completely dissect the disc from the vertebrae using a straight edge razor or cutting tool to insure straight, parallel cuts (Korecki et al. 2007), the current procedure maintains the cartilaginous and/or vertebral endplates as part of the disc organ system. This technique creates a more anatomically complete structure; it inhibits aberrant swelling, maintains collagen connectivity, and reduces disc cell death. Several investigators have developed improved methods of dissections that allow nutrient transport while retaining the anatomic structure more completely (Gantenbein et al. 2006; Gawri et al. 2011). The overall processes are similar and involve coarse dissection, sterilization and isolation of the disc by dissection, thorough cleaning of the vertebral endplates, and eventual placement in a bioreactor system.
22.3.5.2 Procedures for Cleaning the Endplate
The procedures described by Gantenbein et al. (2006) used intervertebral discs obtained from experimental sheep (used primarily for an alternate experiment) that were heparinized prior to death, making cleaning of the endplates relatively easy. After dissection, residual blood within the endplate was aspirated with a Pasteur pipette and using a syringe with an 18-gauge needle and a jet of phosphate-buffered saline. Since the availability of large experimental animals is limited and obtaining only the tail can be costly both economically and ethically, it was necessary to develop methods for cleaning vertebral endplates that were effective for animals that were not heparinized prior to death, thus creating the possibility of using bovine tails which are easily obtained from the abattoir. Different cleaning procedures are available to clean the vertebral capillaries and enable diffusion through the endplates. Our protocol for removal of clotted blood is described in Box 22.2.
An alternate procedure used for organ culture loading involves removal of vertebral endplates and retention of just the cartilaginous portion of the plate. Instead of cleaning the residual ossified vertebral tissue, the discs are further processed by surgically ablating the vertebral bone with a surgical round-end bit attached to a high-speed drill, until the cartilage surface is exposed (Gawri et al. 2011; Jim et al. 2011). Intervertebral discs with cartilage endplates and no calcified bone can be maintained in culture or loaded into a bioreactor (Haglund et al. 2011). It is notable that for the dissections that retain the cartilage endplates, the loading surfaces are not straight and parallel, so loading platens that accommodate the unique curvature and size of the cartilaginous EPs are required (Haglund et al. 2011) and as such are a challenge for precise loading.
22.3.5.2.1 Box 22.2 Refined Dissection Procedures for Isolation of Disc Explants with Vertebral Endplates
The whole tail should be disinfected before dissection. To access the discs, soft tissue should be removed from the caudal spine.
For better accessibility, the spinous and transverse processes of the vertebrae can be removed. In our laboratory, we use a simple histological saw. After removal of surrounding tissue, muscles, nerves, and blood vessels should be removed carefully to avoid cutting into the disc.
To make exact cutting possible, the vertebrae should be rough-cut approximately in half to facilitate the successive precision cutting.
A high precision histological band saw (e.g., Exakt Apparatebau, GmbH, Norderstedt, Germany) should be used to make two parallel transverse cuts through the vertebral endplate just proximal and distal to the cartilage (1–2 mm adjacent to the disc; Fig. 22.4a).
Fig. 22.4
Dissection procedure for cultures with vertebral endplates. (a) Isolation of the intervertebral discs from vertebrae; (b) growth plate removal; (c) intervertebral discs bone-covered endplate cleaning
22.4 Dynamic Compression Loading Models
The addition to dynamic compression loading to intervertebral disc cultures with retained bone-covered endplates provided another significant technical advance in organ culture techniques (Jünger et al. 2009). This method comprised a pneumatically actuated bioreactor loading system capable of loading and culturing whole intervertebral discs with bone endplates and able to simultaneously apply compression loads to 4 individually controlled discs. Physiological loading was simulated with a rest phase at force equivalent of 0.2 MPa for 8 h (representing sleep), an active phase of 0.6 MPa for the remaining 16 h (to simulate time awake), and 2 simulated bouts of exercise lasting 4 h at 0.2 Hz and ±0.2 MPa (i.e., spanning from 0.4 to 1.0 MPa during the active phase). These simulated physiological loading cycles proved beneficial to ovine disc explant cultures as there were no changes in metabolic activity observed after 3 weeks of culture (Jünger et al. 2009). The design specifications of the chambers and pneumatic actuator were optimized for culturing relatively small discs with a diameter of ∼15 mm (Fig. 22.5a).
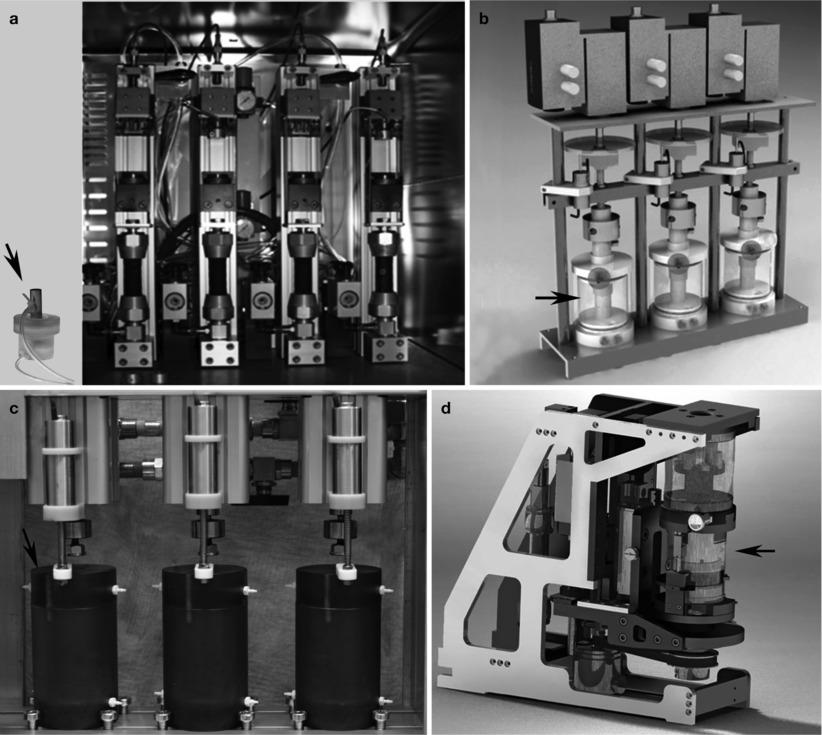
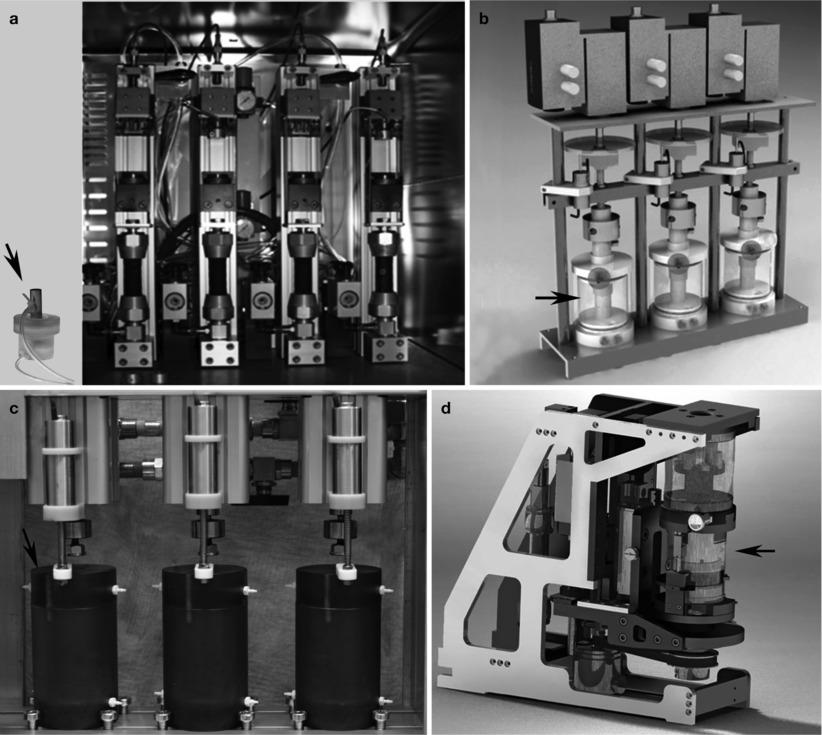
Fig. 22.5
Computer-controlled organ culture loading devices. (a) Pneumatically actuated multi-chamber system for axially loading intervertebral discs of medium size (up to 15 mm in diameter) (system described in Jünger et al. 2009). (b) Pneumatically actuated multi-chamber system for axially loading of bovine or human discs (Figure modified from Haglund et al. 2011). (c) Hydraulically actuated multi-chamber system for applying high magnitudes of axial loading on bovine or human intervertebral discs (Ben Walter and James Iatridis). (d) Pneumatically actuated device for axial and torsional loading on bovine discs (With kind permission of Benjamin Gantenbein-Ritter; system described in Walser et al. 2012). All of these loading devices incorporate mechanical parts including a displacement sensor, a load sensor, and a pneumatic or hydraulic actuator
Haglund et al. (2011) developed an organ culture loading device for culturing intervertebral discs with cartilage endplates (based on the dissection technique described by Gawri et al. (2011)) for 4 weeks. During the first week, the discs were cultured without external load, followed by 2-day static load (0.1 MPa) and 19 days with a dynamic loading regimen consisting of 0.1 MPa static loading with 2 periods of sinusoidal dynamic loading between 0.1 and 0.3 MPa load at 0.1 Hz for 2 h separated by a 6-h 0.1 MPa static load period (Haglund et al. 2011). In this model, cell viability was maintained for up to 4 weeks, and Western blot analysis revealed that the dynamic loading regimen preserved intact aggrecan and prevented the increase in degraded aggrecan that was observed under free-swelling culture conditions. The culture chambers were designed to accommodate discs between 20 and 60 mm, making them sufficient in size for bovine and human intervertebral discs (Fig. 22.5b, Haglund et al. 2011). Another multi-chamber organ culture device is being developed by our group that is capable of dynamically loading bovine coccygeal or human lumbar discs in organ culture in axial compression under relatively high loading conditions. The device is designed to fit in an incubator; it is hydraulically actuated, controlled via proportional solenoids, and capable of applying high forces required for large human lumbar intervertebral discs (up to ∼5.5 kN; ∼2 MPa; Fig. 22.5c). An additional device under development allows loading both in axial compression and in torsion so that the explants can be loaded in multiple degrees of freedom simultaneously (Fig. 22.5d; Walser et al. 2012).
Overall, organ culture has evolved from the first studies, where intervertebral discs were simply placed in culture media and allowed to free swell, to the current elegant computer-controlled systems capable of loading several disc organs simultaneously, in multiple degrees of freedom. The major advances involved controlling swelling, retaining endplates to allow nutrient transport, and applying physiological loads. The next sections describe the usage of these models for degenerative and regenerative studies.
Organ culture permits the investigation of concurrent changes in both structure and cellular responses in a specific and controlled environment. As these models are well controlled, they can be used to determine how multiple factors individually and together contribute to changes in the intervertebral disc biology and degeneration. These organ culture models have been used to address the following scientific questions:
1.
What factor(s) causes and/or contributes to pathogenesis of disc degeneration?
2.
What potential therapeutic approaches can be used to prevent and/or slow the degeneration process?
3.
Can human intervertebral discs be maintained in culture? Research on human tissues addresses issues most directly relevant to health.
22.5 Insights into Mechanism of Degeneration Using Disc Organ Culture
Intervertebral disc degeneration has been defined as “an aberrant, cell-mediated response to progressive structural failure” (Adams and Roughley 2006) with multiple factors thought to be involved in its initiation and progression. The role of these factors is reviewed in other chapters of this book. The majority of current degeneration models are induced using mechanical, structural, nutritional, or inflammatory challenges. This work is providing an improved understanding of individual and interacting factors that cause or lead to degenerative disc disease.
22.5.1 Altered Media Conditions
When compared with in vivo studies that are directed at assessing nutrient transport and uptake by the tissues of the disc, organ culture models provide a much simpler approach to elucidating the importance of this confounding factor. The relevance of nutrient transport as a predisposing factor was demonstrated using nucleus pulposus cell culture (Bibby et al. 2005; Horner and Urban 2001). However, some in vivo models have failed to show that limited nutrition induces degeneration (Hutton et al. 2004; Krebs et al. 2007), possibly due to the lack of precise control over chemical or nutritional boundary conditions. A whole-organ culture study in which there was excellent control over nutrient levels was able to address this controversy (Jünger et al. 2009). Jünger et al. (2009) cultured intervertebral discs under limiting glucose conditions and found that low glucose concentrations (2 g/l vs. 4.5 g/l in normal media) reduced the viability of ovine caudal discs by 40–50 %. However, they also noted that the remaining viable cells exhibited no changes in cellular metabolism, suggesting that limited nutrition alone was not sufficient to initiate a degenerative cascade.
Pro-inflammatory cytokines, specifically tumor necrosis factor-α (TNFα) and interleukin-1-β (IL-1β), are associated with increased degeneration (Le Maitre et al. 2005). Since both cytokines are known to induce catabolic shifts in gene expression and influence the activation of proteases (Hoyland et al. 2008; Seguin et al. 2005), these pro-inflammatory cytokines have served as an attractive target for studies of the degenerative process. Bovine discs that were treated with media containing 200 ng/ml of TNFα for 7 days experienced dramatic losses of aggrecan and altered gene expression that continued following cytokine removal (Walter et al. 2012). Thus, from a mechanistic viewpoint, the effects of injury may be directly linked to upregulation of this and other cytokines.
22.5.2 Mechanical and Nutritional Challenges
Mechanical loading is known to alter metabolic activity and matrix integrity (Iatridis et al. 2006; Walsh et al. 2000), and hence a threshold of “healthy” loading has been described in which immobilization (or underloading) and overloading can both lead to deleterious or degenerative changes (Stokes and Iatridis 2004). Immobilization reduces anabolic metabolism, and overloading can induce micro-injuries (or even larger injuries) which can accumulate and contribute to the degenerative process.
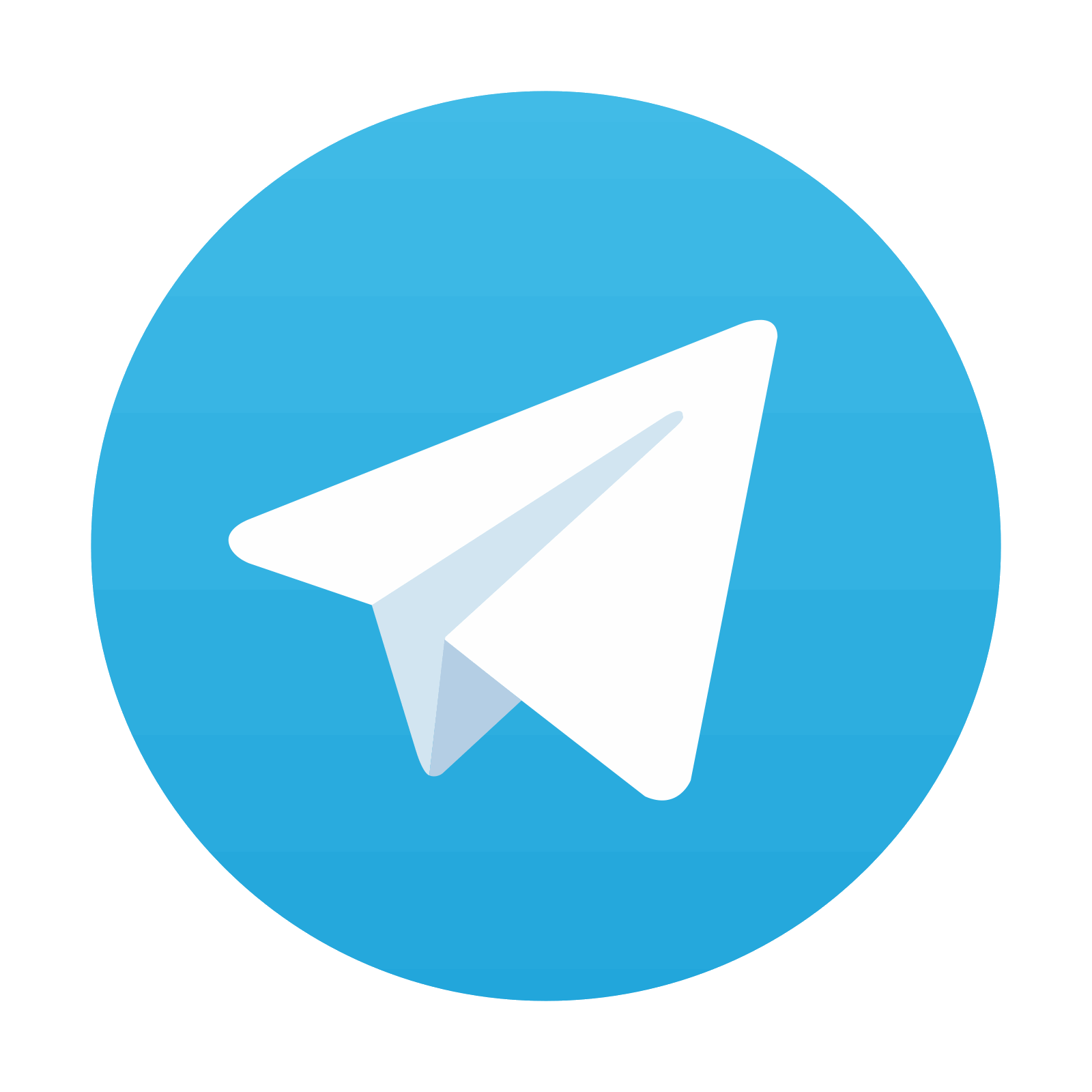
Stay updated, free articles. Join our Telegram channel
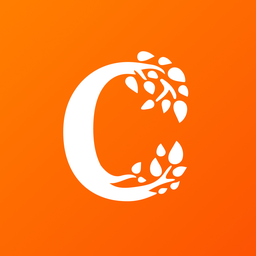
Full access? Get Clinical Tree
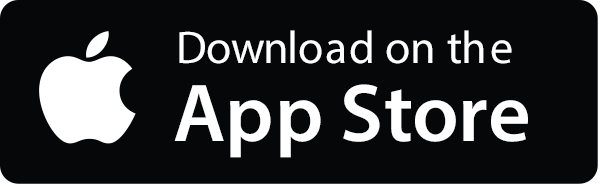
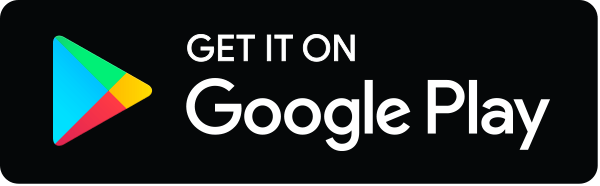