generating motion in three planes, with predominance of the planes of motion in function of the magnitude of the corresponding vectorial component.
The functional capacity of the foot and ankle is age dependent. The functional field is the largest in the newborn and it gradually constricts with aging, more in the transverse segment than in the vertical. At age 2 to 6 years, the field is transversely oval; at age 40 it is converted into a high oval; and, by age 70, it is narrow, limited mainly to the vertical segment.8 In terms of functional capacity, the foot at age 70 years can dorsiflex and plantarflex but has a limited capacity to adapt to walking on uneven ground.
position of the talus (Figs. 10.8 and 10.9). Barnett and Napier11 based their conclusions on the determination of the curvatures of the lateral and medial marginal profiles of the talar trochlea. The center of the curvature being the axis of motion, the lateral profile is “almost always an arc of a true circle and in all positions of the talus the axis of rotation must pass through the center of this circle.’11 The medial profile is formed by the arcs of two circles with different radii. The arc of a small circle, occupying the anterior one third of the medial profile, corresponds to the dorsiflexion arc; the center of the circle is high in location. The arc of a large circle, occupying the posterior two thirds of the medial profile, corresponds to the plantar flexion arc; the center of the circle is low in location.
of plantar flexion and dorsiflexion of the ankle.17 The markings were not parallel and converged toward a point 10.6 to 12.7 cm (4 to 5 inches) medial to the ankle joint and the talar trochlea offered at any time to the bimalleolar fork not the transverse dimension but a larger and constant generating line of the truncated cone (Fig. 10.17). The potential play of the talus in plantar flexion is thus absent.
TABLE 10.1 REPORTED NORMAL RANGES OF ANKLE JOINT MOTION | ||||||||||||||||||||||||||||||||||||
---|---|---|---|---|---|---|---|---|---|---|---|---|---|---|---|---|---|---|---|---|---|---|---|---|---|---|---|---|---|---|---|---|---|---|---|---|
|
the tibia. The inferior peroneo-tibial ligaments are relaxed and the synovial fringe is expulsed from the peroneo-tibial interval and appears in the external angle of the mortise.
additional sectioning of the posterior tibiofibular ligament, the total diastasis was 7.3 mm (range, 3.0 to 15.5 mm). The findings with regard to the degree of rotation paralleled those regarding distasis. The mean external rotation increased 2.7 degrees after the anterior tibiofibular ligament was cut. The mean total increase in rotation was 10.2 degrees when all three ligaments were sectioned.
TABLE 10.2 CONTACT AREAS IN INTACT SPECIMENS | |||||||||||||||||||||||||||||
---|---|---|---|---|---|---|---|---|---|---|---|---|---|---|---|---|---|---|---|---|---|---|---|---|---|---|---|---|---|
|
patterns of response to position of the ankle. On the medial side, there was a relatively constant force throughout plantar flexion, up to roughly 5 degrees of dorsiflexion. From there on, there was a rapid decrease in force. Laterally, tibiotalar forces gradually increased as the ankles moved from extreme plantar flexion to 5 degrees of dorsiflexion.” With further dorsiflexion, the response to force flattened out (Figs. 10.19, 10.20, 10.22).
the flexor digitorum longus tendon, the flexor hallucis longus tendon, and their fibrous sheaths contribute to stability.
![]() Figure 10.23 In marked plantar flexion the anterior talofibular ligament (1) braces the talus and makes a turn around the anterolateral corner of the talar body. |
ligament is taut in plantar flexion and less tense in dorsiflexion (Fig. 10.27), whereas in others the tension in this ligament remains constant in all positions.
![]() Figure 10.26 Tension in calcaneofibular ligament (CFL). (A) CFL taut in dorsiflexion of ankle. (B) CFL relaxed in plantar flexion of ankle. |
![]() Figure 10.27 In this anatomic specimen of the ankle, the calcaneofibular ligament (CF) is taut in plantar flexion (A) and less tense in dorsiflexion (B). |
ligament, of the ankle joint, and prevents the talar tilt of the talus. In plantar flexion, the anterior talofibular ligament is vertical and functions as a collateral ligament, stabilizing the talus laterally. The average angle between the two ligaments, measured in their projection on the sagittal plane, is 105 degrees ± 24 degrees.10 The reciprocal arrangement of the two ligaments is efficient if the angle between the two ligaments is 90 degrees. A horizontal calcaneofibular ligament does not provide the same stability.
talofibular ligament and the calcaneofibular ligament was again confirmed. During the arc of extension-flexion, when one ligament is relaxed, the other is strained, and vice versa. This is similar to Inman’s concept of coupling of the two ligaments.
TABLE 10.3 VARIATIONS IN DEGREE OF NORMAL TALAR TILT | ||||||||||||||||||||
---|---|---|---|---|---|---|---|---|---|---|---|---|---|---|---|---|---|---|---|---|
|
TABLE 10.4 NORMAL ANTERIOR TALAR DISPLACEMENT | |||||||||||||||||||||||||||||||||
---|---|---|---|---|---|---|---|---|---|---|---|---|---|---|---|---|---|---|---|---|---|---|---|---|---|---|---|---|---|---|---|---|---|
|
transection of the ligament, and in dorsiflexion the increase was 5.7 degrees ±3.6 degrees, or 62%, from the initial talar rotation of 10.2 degrees ±6.7 degrees. In essence, the release of the anterior talofibular ligament increases the anteromedial shift of the talus and allows a lateral talar tilt.
talus is resisted by the anterior talofibular ligament.42 This ligament is an essential component of the supportive horizontal ligamentous ring (see Fig. 10.42). The external rotation of the leg places the ligament under tension and the excess may lead to its rupture.
to that of the calcaneofibular ligament. These experiments were conducted with the tested ligament oriented near vertically, which corresponds to a position of plantar flexion for the anterior talofibular ligament and a position of dorsiflexion for the calcaneofibular and the posterior talofibular ligaments.
of the superficial or the deep deltoid component resulted in no change in the talar lateral or anterior shift or in the medial tilt. The transection of both the superficial and deltoid ligaments resulted in a medial talar tilt or abduction of 14 degrees (range, 13 to 16 degrees) but without associated increase of the talar anterior or lateral shift (Fig. 10.48).
the foot on the leg without load, the calcaneofibular ligament becomes a major contributor, averaging 65% to stability. The posterior talofibular ligament is a secondary restraint. This ligament provides no resistance in 15 degrees of dorsiflexion. The deltoid ligament and the anterior talofibular ligament have secondary influences. With loading, the calcaneofibular restraint averages 43% and the posterior talofibular ligament 24%. The deltoid ligament now provides substantial support, averaging 20% except in neutral (3.2%), and the anterior talofibular ligament contributes an average of 17% to stability except in plantar flexion (5.3%). The articular surfaces under loading provide an average of 27% of stability (Fig. 10.51).
oriented upward, anteriorly and medially. It penetrates the posterolateral corner of the os calcis, passes perpendicular to the canalis tarsi, and pierces the superomedial aspect of the talar neck. Manter reported the angulation of the axis to have a 42 degrees average inclination (range, 29 to 47 degrees) in the sagittal plane relative to the horizontal line and a 16 degrees average medial deviation (range, 8 to 24 degrees) in the transverse plane relative to the long axis of the foot passing through the first interdigital space.50 Inman provided measurements that are very similar: 42 degrees ± 9 degrees of inclination in the sagittal plane and 23 degrees ± 11 degrees of medial deviation in the horizontal plane relative to the axis of the foot passing through the second interdigital space (Fig. 10.53).10
surface as a female ovoid surface. The combined anterior and middle calcaneal surfaces form a female ovoid surface and the inferior articular surface of the talar head forms a male more or less flattened ovoid surface. MacConaill and Basmajian analyzed the motion components generated by the moving ovoid surfaces relative to each other.30 A male ovoid surface moving on a female ovoid surface slides, rolls, and spins. The rolling is in a direction opposite to the sliding (Fig. 10.62). A female ovoid surface moving on a male ovoid surface slides, rolls or rocks, and spins. The rolling is in the direction of sliding (Fig. 10.63). The roll is a tilt that maintains the surface contact and the spin maximizes the congruency. Huson analyzed the spin at the posterior talocalcaneal joint.8 Because of the differential of the curvatures of the articular surface—more curved medially and less curved laterally—a pure sliding creates more incongruency of the corresponding surfaces, whereas an associated spin minimizes the incongruency (Fig. 10.64). This interpretation is inclusive in the broader and more comprehensive analysis of the movement of ovoid surfaces presented by MacConaill and Basmajian.30 If the ovoid surfaces are obliquely oriented with regard to the long axis of the foot, the generated motion will have two components. The associated spin creates the third motion component.
TABLE 10.5 REPORTED RANGES OF SUBTALAR MOTION | ||||||||||||||
---|---|---|---|---|---|---|---|---|---|---|---|---|---|---|
|
![]() Figure 10.63 A female ovoid surface moving on a male ovoid surface slides, rolls, and spins. The rolling is in the direction of the sliding. |
talocalcaneal interosseous ligament of the canalis tarsi and the cervical ligament may be considered as cruciate ligaments of the subtalar joint, as determined by their opposite orientation. The cervical ligaments and the calcaneofibular ligament have an approximate similar orientation.
degrees. With internal rotation force applied, calcaneotibial rotation increased from plantar flexion to neutral ankle position. With external rotation force applied, calcaneotibial external rotation from neutral to maximal dorsiflexion increased. Their conclusion was that “the ankle is less stable in plantarflexion when inversion and internal rotation forces are applied” and “the ankle was less stable in dorsiflexion when eversion and external rotational forces were applied.”
of these functional discrepancies.32 Furthermore, the functional implication of the variable anatomic relationship between the calcaneofibular ligament and the lateral talocalcaneal ligament was investigated by Trouilloud and colleagues in 26 ankles.61 They divided their specimens into three types: A, B, and C. In type A (35%), a lateral talocalcaneal ligament blends with or reinforces intimately the calcaneofibular ligament and diverges from the latter at the talar or at the calcaneal insertion. In type B (23%), a distinct lateral talocalcaneal ligament is present just anterior to the calcaneofibular ligament. In type C (42%), the lateral talocalcaneal ligament is absent.
of inversion assessment were used: manual and roentgenographic. Under inversion load with the calcaneofibular ligament intact the mean elongation of the cervical ligament was 0.58 mm ±0.33 mm by manual measurement and 0.46 mm ± 0.23 mm by x-ray measurement. With the calcaneofibular ligament transected, under inversion load, the elongation of the cervical ligament was 0.88 mm ±0.37 mm by manual measurement and 0.78 mm ±0.37 mm by x-ray measurement. With the transection of the calcaneofibular ligament, the inversion range of motion increased 7.5 degrees ± 2.75 degrees manually and 7.7 degrees ±2.95 degrees by x-ray measurement.
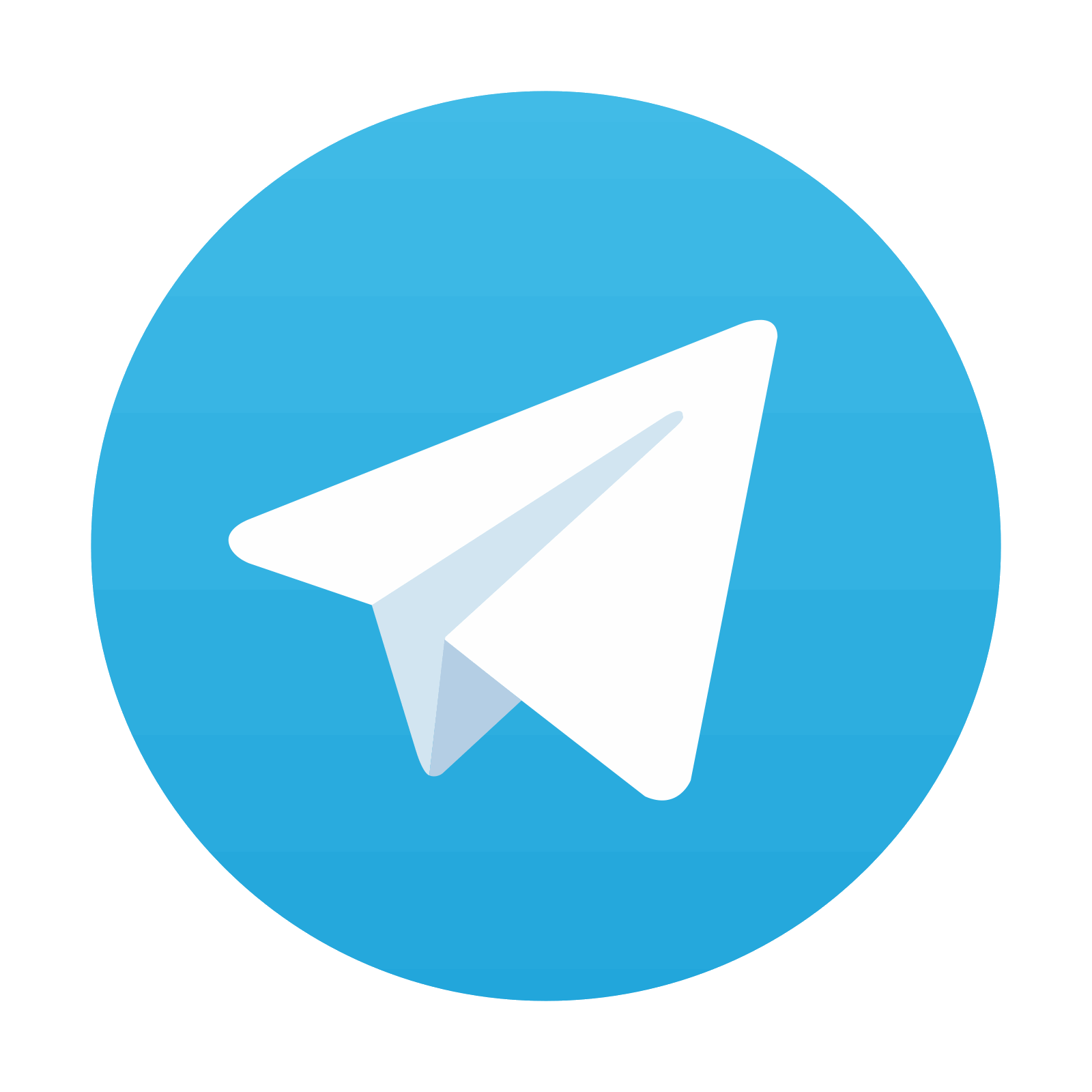
Stay updated, free articles. Join our Telegram channel
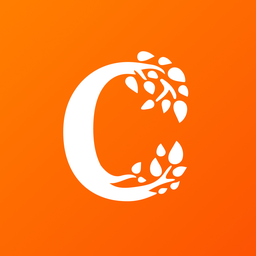
Full access? Get Clinical Tree
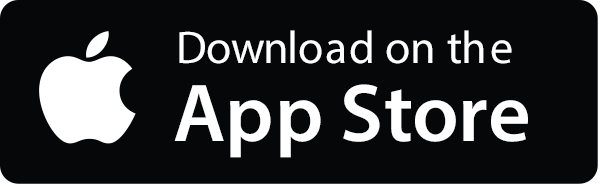
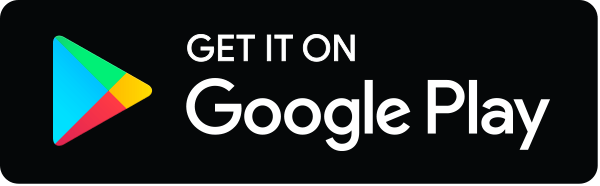