Scaffold
Targeted issues
Administration
Ref.
Vascularized iliac bone graft
Osteonecrosis of femoral head
Implanted
Iliac bone graft
Osteonecrosis of femoral head
Porcine-derived collagen type I/III membrane
Knee cartilage defects
Collagen
Osteochondral lesions of the talus
[9]
Hyaluronan-based scaffolds
Articular chondral lesions
[10]
Sodium hyaluronate
Meniscus regeneration
Injected
[11]
Fibrin glue
Knee cartilage defects
[12]
Demineralized bone matrix (DBM)
Distal tibial fractures
[13]
Furthermore, cellular approaches play an important role in bone and cartilage tissue engineering and regenerative medicine (TERM). The existence of several different cell culture procedures (e.g., cell types, cell isolation, expansion and seeding, and pre-culture conditions before in vivo assays) may have an impact on the experimental outcomes. The present chapter provides an overview of in vitro and in vivo preclinical strategies in bone and cartilage TERM to achieve more standardized culture conditions for future studies and hence enhance bone and cartilage formation. Current bone and cartilage repair options that utilize implanted cells are limited by the number of cells available for isolation and by their uncontrolled phenotypic alterations. As such, different cell types have been investigated as cell sources for bone and cartilage engineering due to their well-established ability to generate bone and cartilage-like ECM under the appropriate culture conditions (Table 49.2).
Table 49.2
Origin and source of human stem cells applied in bone and cartilage tissue engineering
Cell origin | Cell source | Targeted tissues | Ref. |
---|---|---|---|
Autologous | Adult human peripheral blood CD34+ cells | Bone | [4] |
Chondrocytes | Cartilage | ||
Synovium-derived mesenchymal stem cells (S-MSCs) | |||
Cortical bone fragment-derived mesenchymal stem cells (CBF-MSCs) | Bone | [18] | |
Bone marrow-derived mesenchymal stem cells (BM-MSCs) | Bone and cartilage | ||
Adipose tissue-derived mesenchymal stem cells (AT-MSCs) | |||
Peripheral blood-derived mesenchymal stem cells (PB-MSCs) | |||
Dental pulp-derived mesenchymal stem cells (DP-MSCs) | |||
Adipose-derived stromal vascular fraction (SVF) | |||
Allogenic | Bone marrow-derived mesenchymal stem cells (BM-MSCs) | [11] | |
Embryonic stem cells (ESCs) | [33] | ||
TGF-β1 modified human chondrocytes | |||
Umbilical cord blood mesenchymal stem cells (CB-MSCs) | |||
Induced pluripotent stem cells (iPSCs) |
49.2 Preclinical Cell Culture Methods
49.2.1 Cell-Based Methods
49.2.1.1 Human Mesenchymal Stem Cell Culture
To restore bone and cartilage defects in clinical applications, some critical issues that are inherently related to the culture conditions must be considered. Human AT-MSCs (also called hASCs) can be obtained from lipoaspirates, which is a less invasive procedure compared to the harvesting of human BM-MSCs, which requires drilling into the bone. hASCs can differentiate into the chondrogenic, osteogenic, adipogenic, myogenic, neurogenic, and hepatogenic lineages [40]. Moreover, stem cell yields are higher from adipose tissue, with 1 g containing an average of 2 × 106 cells with 10% being ASCs [41]. Therefore, adipose tissue represents an abundant and practical source of multipotent stem cells for autologous and allogenic cell transplantation approaches.
Conventional hASC Isolation and Expansion Protocol
Standard methods for isolating and purifying human ASCs.
Ten grams of adipose tissue was harvested, washed in phosphate-buffered saline (PBS) supplemented with 10% penicillin-streptomycin solution (vol/vol).
After washing, the adipose tissue is minced and digested in 15 mL of 37 °C warmed Dulbecco’s modified Eagle’s medium (DMEM)/collagenase type II from Clostridium histolyticum solution (1:1) supplemented with 10% penicillin-streptomycin solution (vol/vol) while shaking at 37 °C and 180 rpm overnight.
After digestion, filter the samples through a 100 μm cell strainer to a new 50 mL Falcon tube, complete to 20 mL with DMEM, and centrifuge 10 min at 800 × g to allow for complete cell/layer separation.
Aspirate the supernatant, resuspend the pellet in 15 mL of PBS to wash the cells, and centrifuge 10 min at 300 × g.
Remove the supernatant and resuspend the pellet in 5–10 mL of DMEM (volume depending on the size of the pellet obtained).
Count the cells using a Neubauer chamber.
The isolated hASCs were seeded in 75 cm2 culture flasks at a density of 5 × 103 cells/cm2, cultured in DMEM supplemented with 10% of fetal bovine serum (FBS) (vol/vol) and 1% of penicillin-streptomycin solution (vol/vol), and incubated at 37 °C with 5% CO2 (Fig. 49.1).

Fig. 49.1
hASCs cultured in DMEM supplemented with 10% of fetal bovine serum (FBS) (vol/vol) and 1% of penicillin-streptomycin solution (vol/vol) and incubated at 37 °C with 5% CO2; scale bar = 100 μm
Standard Chondrogenic Differentiation Protocol
hASCs at passage 4 (P4) were cultured in the form of pellets (obtained by centrifugation of 5 × 106 cells at 800 × g) in 15 mL Falcon tube.
The pellets were then cultured in chondrogenic media composed of DMEM/F-12 (with glutamine and sodium pyruvate) or DMEM-high glucose (DMEM-HG) supplemented with 1% FBS (vol/vol), 1% of penicillin-streptomycin solution (vol/vol), 100 nM dexamethasone, 1% insulin-transferrin-selenium-X (ITS), 50 μg/mL ascorbate-2-phosphate, 40 μg/mL l-proline, 50 ng/mL insulin-like growth factor 1 (IGF-1), and 10 ng/mL TGF-β1 for 21 days.
The cells were incubated at 37 °C with 5% CO2.
The medium was replaced every 3 days.
After the 21 days, the pellets were collected. Some of them were fixed in 4% neutral buffered formalin and used for chondrogenic histological analysis (Alcian blue, toluidine blue, and safranin O staining). The remaining pellets were used to evaluate the gene expression levels of the chondrogenic markers, collagen II, aggrecan (ACA), and Sox9 by quantitative reverse transcription PCR (RT-qPCR) [45–47].
Standard Osteogenic Differentiation Protocol
hASCs at passage 4 (P4) were seeded in 24-well TCPS plate at a density of 15 × 103 cells/cm2.
The cells were cultured in osteogenic media composed of DMEM or α-MEM supplemented with 1% FBS (vol/vol), 1% of penicillin-streptomycin solution (vol/vol), 100 nM dexamethasone, 10 mM β-glycerol phosphate, and 5 mg/mL ascorbate-2-phosphate for 21 days.
The cells were incubated at 37 °C with 5% CO2.
The medium was replaced every 3 days.
After the 21 days, some of the wells were fixed in 4% neutral buffered formalin and used for osteogenic histological analysis (alizarin red staining). The remaining wells were used to evaluate the gene expression levels of the osteogenic markers, alkaline phosphatase (ALP), osteocalcin (OSC), osteopontin (OPN), and Runx2 by quantitative reverse transcription PCR (RT-qPCR) [45–47].
To be able to use cells clinically, it is required to define good manufacturing practice (GMP) methods to isolate as well as to culture them in a well-defined medium under GMP conditions. A number of different companies together with research laboratories started to develop automated closed devices to standardize parts or even the whole cell isolation process. Oberbauer summarized a survey of currently patented, published, or commercially available enzymatic and nonenzymatic adipose tissue-derived cell isolation systems [48].
Several factors can influence clinical cell culturing, such as cell culture media, medium supplements, cell density, surface coating, detachment enzymes, oxygen conditions, and cell plating density. Nevertheless, the medium used for expansion of the cells represents the most crucial factor [49]. Some concerns do exist with the use of FBS for clinical applications, due to (a) the possibility that FBS contains unsafe contaminants such as prions, viral, and zoonotic agents and (b) its inconsistency from batch to batch.
Furthermore, when hASCs are cultured in a medium comprising animal proteins, a large amount of these proteins is retained in the cytoplasm, which can stimulate an immunologic reaction when transplanted in vivo. Therefore, efforts have been made by several international research groups to develop (a) alternative media either by replacing FBS with human-sourced supplements (such as platelet-rich plasma or human serum) or by identifying defined serum-free formulations consisting of key growth/attachment factors and (b) controlled bioreactor protocols.
49.2.1.2 “Humanized” hASC Culture Protocols
The present chapter will focus on the developed alternatives which will guarantee the safe, economical, and ethical practice of biomedical research, through the elimination of many shortcomings of FBS. These alternatives are based on the use of medium supplemented with human platelet-rich plasma (PRP) or human autoserum and on culture under animal-/xeno-free media conditions.
Platelet-Rich Plasma Supplement
Over the last two decades, human platelet-rich plasma (PRP), an autologous derivative of whole blood, has been widely used as therapy for orthopedic injuries [50]. PRP is rich in growth factors including transforming growth factor β1 (TGF-β1), platelet-derived growth factor (PDGF), insulin-like growth factor (IGF), basic fibroblast growth factor (bFGF), and vascular endothelial growth factor (VEGF) [51].
Platelet-Rich Plasma Preparation
Human PRP was obtained by venipuncture in acid citrate dextrose (ACD) tubes.
Briefly, 10 mL of venous blood was drawn from the antecubital vein in sterile vacuum tube containing 1 mL citrate phosphate dextrose adenine (CPDA) as anticoagulant and centrifuged at 250 × g for 15 min.
After the first centrifugation, two layers were seen clearly. The superior yellow layer was consisting of platelet-rich (PRP) and platelet-poor (PPP) plasma, and lower red layer was consisting of erythrocytes and leukocytes.
Then the complete upper yellow layer was transferred into plain vacuum tube.
After the second centrifugation at 200 × g for 15 min, approximately 2 mL was PRP at the bottom of the vacuum tube and the upper rest was plasma PPP fraction.
The PRP was activated with 10–20% CaCl2, thrombin solution.
Platelet-Rich Plasma Culture Medium Supplementation
Human ASCs will be isolated under GMP conditions.
For basal expansion/growth, hASCs at passage 4 (P4) were seeded in 24-well TCPS plate at a density of 15 × 103 cells/cm2. Then, cells were cultured in DMEM supplemented with 1% penicillin-streptomycin solution and 20% PRP (vol/vol), prepared and activated as previously described. This concentration of PRP offers the optimal condition for cell growth with increased proliferation compared to FBS supplementation [55]. The cells were incubated at 37 °C with 5% CO2. The medium was replaced every 3 days.
For chondrogenic differentiation, hASCs at passage 4 (P4) were cultured in the form of pellets (obtained by centrifugation of 5 × 106 cells at 800 × g) in 15 mL Falcon tube. Then, cells were cultured in chondrogenic media composed of DMEM/F-12 (with glutamine and sodium pyruvate) or DMEM-high glucose (DMEM-HG) supplemented with 20% PRP (vol/vol), 1% of penicillin-streptomycin solution (vol/vol), 100 nM dexamethasone, 1% insulin-transferrin-selenium-X (ITS), 50 μg/mL ascorbate-2-phosphate, 40 μg/mL l-proline, 50 ng/mL insulin-like growth factor 1 (IGF-1), and 10 ng/mL TGF-β1 for 21 days. The cells were incubated at 37 °C with 5% CO2. The medium was replaced every 3 days.
For osteogenic differentiation, hASCs at passage 4 (P4) were seeded in 24-well TCPS plate at a density of 15 × 103 cells/cm2. Then, cells were cultured in DMEM supplemented with 1% penicillin-streptomycin solution (vol/vol), 100 nM dexamethasone, 10 mM β-glycerol phosphate, 5 mg/mL ascorbate-2-phosphate, and 20% of PRP (vol/vol) for 21 days [52]. The cells were incubated at 37 °C with 5% CO2. The medium was replaced every 3 days.
Besides, the clinical advantages of PRP such as (a) its non-stimulative and bioactivity-supporting role, (b) its outstanding cost to benefit ratio, (c) its preparation that does not require expensive devices, and (d) its low risk for infection, the careful preparation, and quality control of PRP, in combination with the rigorous evaluation of recipient implantation sites, will lead to improved clinical outcomes for patients [56].
Human Autoserum-Based Supplement
Autologous human serum was investigated as a substitute for fetal bovine serum in the ex vivo expansion medium to avoid the transmission of dangerous pathogens/contaminants during clinical reconstruction procedures.
Human Autoserum Preparation
Human serum was derived from whole blood donations.
From each donor 400–500 mL of whole blood was drained into blood bags without anticoagulants and allowed to clot for 4 h [57] to overnight [58] at 4 °C.
In some protocols, the blood is quickly transferred to 10 mL vacutainer tubes without anticoagulants and allowed to clot for 2 h at room temperature [59] or 4 h at 4 °C [60].
Afterward, the blood was centrifuged at 1.800 × g for 15 min at 4 °C.
Serum was collected and filtered through 0.2 μm membrane, and sterile aliquots were stored at −20 °C.
Human Autoserum (hAS) Culture Medium Supplementation
Human ASCs will be isolated under GMP conditions.
For basal expansion/growth, hASCs at passage 4 (P4) were seeded in 24-well TCPS plate at a density of 15 × 103 cells/cm2. Cells were cultured in DMEM supplemented with 1% penicillin-streptomycin solution (vol/vol) and 10% hAS (vol/vol). The cells were incubated at 37 °C with 5% CO2. The medium was replaced every 3 days [59].
For chondrogenic differentiation, hASCs at passage 4 (P4) were cultured in the form of pellets (obtained by centrifugation of 5 × 106 cells at 800 × g) in 15 mL Falcon tube. Then, cells were cultured in chondrogenic media composed of DMEM/F-12 (with glutamine and sodium pyruvate) or DMEM-high glucose (DMEM-HG) supplemented with 10% hAS (vol/vol), 1% of penicillin-streptomycin solution (vol/vol), 100 nM dexamethasone, 1% insulin-transferrin-selenium-X (ITS), 50 μg/mL ascorbate-2-phosphate, 40 μg/mL l-proline, 50 ng/mL insulin-like growth factor 1 (IGF-1), and 10 ng/mL TGF-β1 for 21 days. The cells were incubated at 37 °C with 5% CO2. The medium was replaced every 3 days.
For osteogenic differentiation, hASCs at passage 4 (P4) were seeded in 24-well TCPS plate at a density of 15 × 103 cells/cm2. Then, cells were cultured in DMEM supplemented with 1% penicillin-streptomycin solution (vol/vol), 100 nM dexamethasone, 10 mM β-glycerol phosphate, 5 mg/mL ascorbate-2-phosphate, and 10% of hAS (vol/vol) for 21 days [59]. The cells were incubated at 37 °C with 5% CO2. The medium was replaced every 3 days.
Nevertheless, the use of hAS showed arguments such as high costs and the high likelihood of factors in the blood of donors, which may dominantly impact on hASC growth, greatly promoting the development of serum-free, chemically defined media.
Serum-Free/Xeno-Free Medium-Based Protocol
Alternatives for FBS have been previously described, such as platelet-derived supplements and human autoserum (hAS). However, restrictions related to the use of serum or its components exist, such as batch variation with serum-supplemented media performance, affecting proliferation rate and differentiation potential. Therefore, defined serum-free (SF)/xeno-free (XF) media formulations have been developed in order to enhance significantly the safety and quality of the transplanted stem cells [61]. These chemically defined media presented some advantages, namely, their precise chemical composition and the absence of xenogeneic contaminants (i.e., microorganisms). Nevertheless, the optimal medium composition mostly depends on the requests of individual experimental conditions and may vary largely between and within cell types.
There are several commercially available SF/XF media for hASC expansion and growth including FibroLife® medium (LifeLine®), MesenCult™-XF Medium (StemCell™), mesenchymal stem cell growth medium DXF (PromoCell), and StemPro® MSC SFM XenoFree medium (Life Technologies) [62].
Human ASCs will be isolated under GMP conditions.
For basal expansion/growth, hASCs at passage 4 (P4) were seeded in 24-well TCPS plate at a density of 15 × 103 cells/cm2. Cells were cultured in SF/XF media supplemented with 1% penicillin-streptomycin solution (vol/vol). Depending on the SF/XS medium manufacturer’s guidelines, other supplements might be added. The cells were incubated at 37 °C with 5% CO2. The medium was replaced every 3 days.
For chondrogenic and osteogenic differentiation, several specific media are commercially available such as Mesenchymal Stem Cell Chondrogenic and Osteogenic Differentiation Medium (PromoCell), StemPro® Chondrogenesis and Osteogenesis Differentiation Kit (Life Technologies), OriCell (Cyagen—for chondrogenic differentiation), and hMSC Chondrogenic and Osteogenic Differentiation BulletKit™ Medium (Lonza).
For chondrogenic differentiation, hASCs at passage 4 (P4) were cultured in the form of pellets (obtained by centrifugation of 5 × 106 cells at 800 × g) in 15 mL Falcon tube. Then, cells were cultured in xeno-free chondrogenic medium supplemented with 1% of penicillin-streptomycin solution (vol/vol) for 21 days. The cells were incubated at 37 °C with 5% CO2. The medium was replaced every 3 days [63, 64].
For osteogenic differentiation, hASCs at passage 4 (P4) were seeded in 24-well TCPS plate at a density of 15 × 103 cells/cm2. Then, cells were cultured in xeno-free osteogenic medium supplemented with 1% penicillin-streptomycin solution (vol/vol) for 21 days. The cells were incubated at 37 °C with 5% CO2. The medium was replaced every 3 days [63, 64].
49.2.2 3D-Based Protocols
Three-dimensional (3D) matrices have been studied to improve cell expansion through the delivery of an active surface for cells to attach and to proliferate. This method provides main advantages such as: (a) an increased surface to volume ratio, thus allowing the growth of cells within a confined volume space; (b) for adherent-dependent cells, the surface properties can be functionalized to improve attachment and proliferation; and (c) 3D surfaces increase the possibilities of providing a microenvironment that can control cell behavior and function by engineered biomaterials and cell niches, naturally seen in in vivo physiology. The following four methods are presently applied for adherent cell-based expansion for clinical trials and commercial production.
49.2.2.1 Scaffolds
Current approaches for bone and cartilage repair are centered on the use of scaffolds providing a suitable three-dimensional (3D) environment supporting the growth of a repair tissue. These 3D structures are often critical, both in vitro and in vivo, to summarizing the in vivo milieu and allowing cells to modulate their own microenvironment. The ideal scaffolds for bone and cartilage tissue engineering must be based on the following basic requirements: porous, biocompatible, biodegradable, and appropriate for cell attachment, proliferation, and differentiation. Several biomaterials have been studied for cartilage and bone tissue engineering [65, 66].
For cartilage tissue engineering, a hydrogel-based approach has been developed in order to produce a new advanced therapy medicinal product (ATMP), where undifferentiated hASCs encapsulated within a novel hydrogel based on methacrylated gellan gum (GG-MA) and cultured with or without chondrogenic induction for 21 days will be able to develop cartilage-like tissue (Fig. 49.2).


Fig. 49.2
Live/dead assay. Calcein-AM (green) and propidium iodide (red) stainings of GG-MA loaded with hASCs 7 days, 14 days, and 21 days after cell encapsulation; scale bar = 200 μm
For cell encapsulation into GG-MA hydrogel, the following protocol will be used:
Human ASCs at passage 4 (P4) were expanded in xeno-free media supplemented with 1% penicillin-streptomycin solution (vol/vol) until sub-confluency.
When reaching 80% confluence, cells were trypsinized using TrypLE Express solution.
Add the required amount of GG-MA with 9 mL of warm, sterile distillated water.
Allow it to dissolve in 37 °C water bath.
Prepare a cell suspension of 3 × 106 cell in 300 μL of cell culture xeno-free media supplemented with 1% penicillin-streptomycin solution (vol/vol).
Add 2.7 mL of GG-MA hydrogel to the cell suspension and quickly mix by pipetting up and down (hydrogel concentration is 2% w/V, cell density is 1 × 106 cell/mL).
Pipette 50 μL of cellular hydrogel to each well.
Cover the hydrogel with 500 μL of basal culture xeno-free medium or xeno-free chondrogenic medium, both supplemented with 1% penicillin-streptomycin solution (vol/vol) for 21 days.
Incubate the tridimensional culture at 37 °C with 5% CO2. The medium was replaced every 3 days.
After the time of incubation, this new ATMP will be administrated into the cartilage defect in vivo [67].
For bone and osteochondral tissue engineering, different biomaterials have been investigated with some of them going to clinical trials [68–70]. In vitro, the following protocol for the cell seeding might be applied:
Sterile scaffolds should be pretreated to prevent air bubble formation in the pores, which can inhibit cell adhesion, through the use of a 20 mL sterile syringe with an attached 21G sterile needle.
Before the cell seeding, the scaffolds were hydrated in culture medium overnight in the CO2 incubator at 37 °C.
Afterward, the scaffolds were removed from the medium and placed into a 48-well suspension cell culture plate.
Seed the hASCs at passage 4 (P4) onto the surface of the scaffolds at different cell densities (e.g., 5 × 102 cells/scaffold, 1 × 103 cells/scaffold, 5 × 103 cells/scaffold, and 1 × 104 cells/scaffold).
Then the scaffolds with cells were kept in the CO2 incubator at 37 °C.
After 3h, the constructs were transferred to a new 48-well suspension culture plate, and each construct was supplemented with 1 mL of culture medium.
Incubate the tridimensional culture at 37 °C with 5% CO2. The medium was replaced every 3 days.
49.2.3 Bioreactors
Large-scale hASC manufacturing should rely on effective and economical GMP-compliant processes that were able to strongly generate cells with well-defined characteristics in quantities that meet clinical demands. These procedures should offer consistent optimal growth conditions for hASC, complete monitoring, and control of culture conditions, while presenting easy scalability. To encounter these supplies, stirred bioreactors offer substantial advantages over static systems. In a static cell culture condition, the environment is continuously changing through the accumulation of metabolites, nutrient depletion from the culture medium, and the resultant changes in pH. This is evidently not representative with the situation in vivo, where cells are continuously in equilibrium between the constant elimination of products and supply of nutrients. In tissue engineering (TE), bioreactors might be used for different purposes: (a) culture/growth of cells (generally in suspension) prior seeding onto the scaffold biomaterial and (b) to achieve specific physical stimulation of the TE construct, in order to support cell/tissue differentiation. The advantages of bioreactors on cell expansion and tissue maturation have been clearly demonstrated [71].
Therefore, stirred bioreactors allow a more homogeneous culture environment and guarantee monitoring and control of key culture factors (e.g., pH, temperature, oxygen contain), while reducing culture handling and costs [72, 73].
Efficient expansion of hASC using a plastic microcarrier-based culture system under xeno-free conditions in spinner flasks has been demonstrated [74]. The protocol used was:
Use of Bellco spinner flasks with a working volume of 80 mL, equipped with 90° paddles (normal paddles) and a magnetic stir bar.
The initial hASC density used was 5 × 104 cells/mL.
Plastic microcarriers were coated with a CELLstart CTS solution (diluted 1:100 in PBS with Ca2+ and Mg2+) for 2 h at 37 °C, with an intermittent agitation (1 min at 300 rpm, 10 min non-agitated) using a Thermomixer comfort, and afterward equilibrated in pre-warmed MesenPRO RS/StemPro MSC SFM XenoFree medium.
hASC, previously expanded under xeno-free static conditions for two passages, was seeded on 20 g/L of pre-coated plastic microcarriers in 15 mL of the respective medium for 30 min, at 37 °C and 5% CO2, with gentle agitation every 5 min.
Then, pre-warmed medium was added until reaching half of the final volume, and the cell suspension was transferred to the spinner flask and submitted to an intermittent agitation regimen (15 min at 25 rpm followed by 2 h non-agitated) during 24 h.
After the initial 24 h, agitation was set at 40 rpm.
After 3 days of culturing, 25% of the medium volume was renewed daily.
Moreover, hASC demonstrated efficient expansion using a controlled stirred-tank bioreactors [75].
The following protocol was used:
After 4 days in the spinner flasks, the microcarrier-cell suspension was transferred to a 1300 mL stirred tank bioreactor equipped with a 3-blade pitched impeller (blades pitched 4° to vertical) and dissolved oxygen and pH probes.
The addition of CO2 was made through gentle sparging from the base of the reactor, and 1.0 M of NaHCO3 was used to maintain pH of culture.
Xeno-free culture medium was added to reach the working volume of 800 mL.
The culture parameters were set to pH 7.2, continuous agitation at 60 rpm, and aeration. According to the experimental setup, dissolved oxygen was set to 9 or 20% of air saturation.
49.2.3.1 Bioreactors Available for Cartilage TE
The improvement of cartilage engineering through the use of bioreactors is well demonstrated [76–78]. To overcome limitations resulting from the mass transport of nutrients into the core of large scaffolds that hereafter affected the extent to which such constructs could mimic native cartilage, more complex bioreactors have been designed i.e., spinner flasks, rotating wall vessel bioreactors, and flow perfusion bioreactors [77]. Recently a study provided a highly efficient and stable bioreactor system for improving in vitro 3D cartilage regeneration and thus will help to accelerate its clinical translation [79].
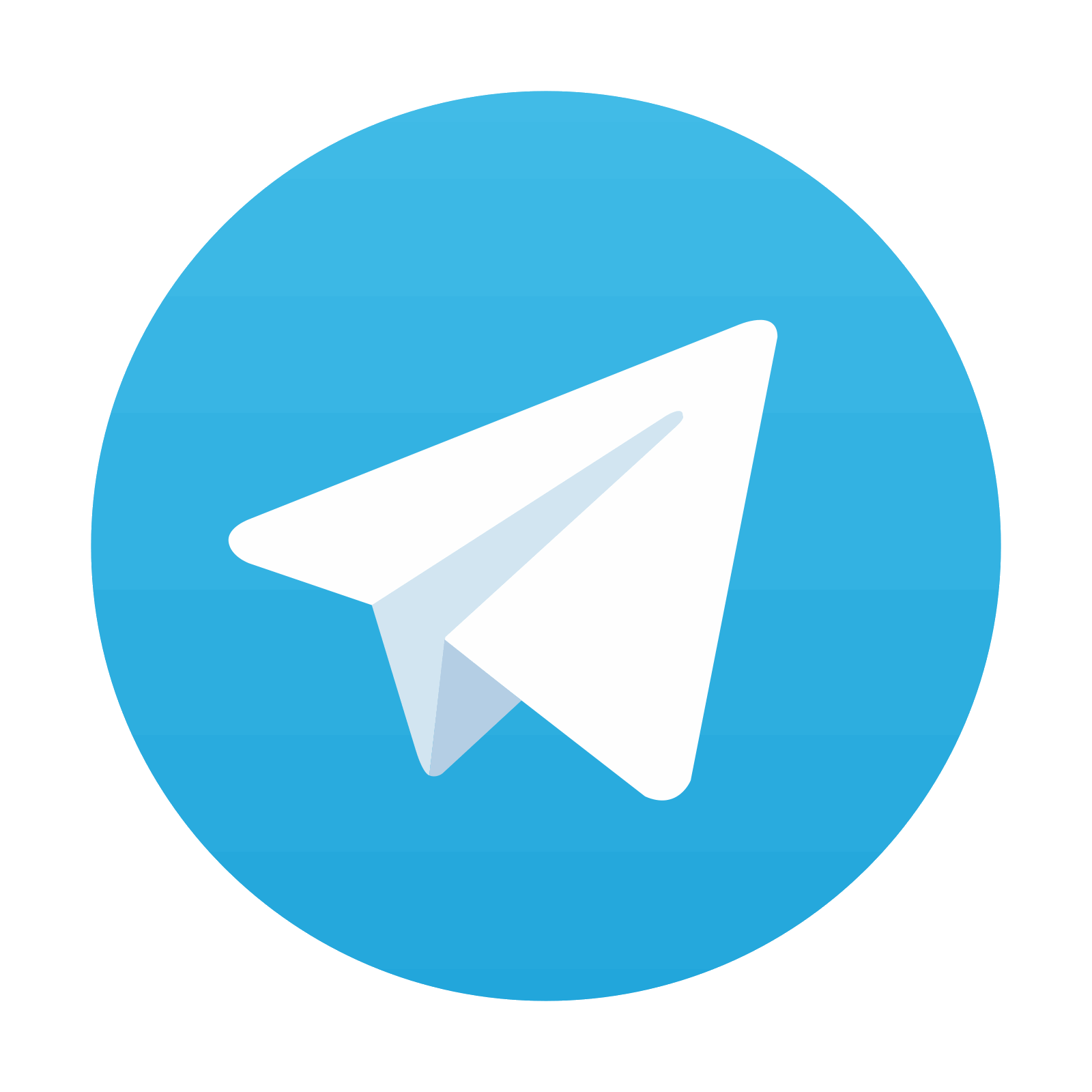
Stay updated, free articles. Join our Telegram channel
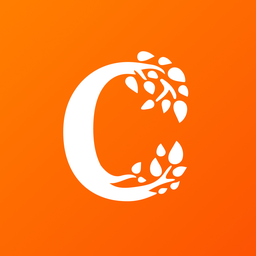
Full access? Get Clinical Tree
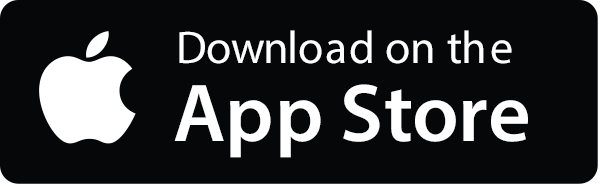
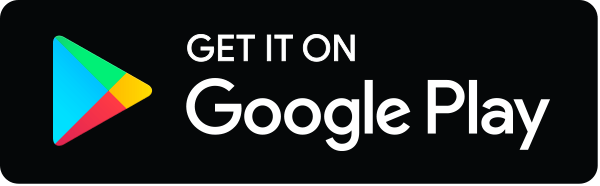