Biomaterials: Metals and Other Nonabsorbables
Sean T. Grambart
Jeffrey C. Christensen
Implantable biomaterials are diverse in physical properties and widely used in musculoskeletal surgery to perform reconstructive and joint replacement surgery. The purpose of this chapter is to review the various materials available to facilitate evaluation of the surgical products that are fabricated with these materials. The physical properties of the material have to match the mechanical stresses placed on the implant, or failure of the implant may occur. Understanding the basic characteristics of the materials is important in the surgical success of implantation of surgical devices. There are a variety of terms used when describing the physical characteristics of biomaterials, especially terms pertaining to mechanical properties. A glossary of terms has been provided at the end of this chapter to provide the reader with a source of technical terms often used by mechanical engineers when characterizing biomaterials.
METALS
By design, metals used to fabricate bioimplants usually contain one main element and a combination of several other materials. These composite metals differ according to their micro-structure, which is a result of the processing method and the elements combined. The mechanical properties, resistance to corrosion, and other properties result from the combination of the composition and the microstructure. In addition, sound manufacturing processes and quality control can help improve the strength and reduce fatigue resistance by decreasing the amount of defects and impurities in the metal.
The discussion of mechanical properties of biometals is often centered on the stress-strain diagram (Fig. 2.1). Metals vary in terms of mechanical stiffness, fatigue resistance, and corrosion resistance, properties with which the surgeon should be familiar.
STAINLESS STEEL
The American Society of Testing and Materials recommends 316L type stainless steel for improved corrosion resistance, wear, and fatigue resistance for implant fabrication. The “L” indicates low carbon content. Chromium is the major component in 316L stainless steel followed by nickel and molybdenum (Table 2.1). A type of austenitic stainless steel, 316L stainless steel is typically hardened by cold working rather than using heat treatment. Heat treatment yields a softer material, whereas cold working increases strength and hardness. The metal can be further modified by an annealing process (i.e., most reconstruction plates are annealed to improve ductility). Surface modification techniques such as anodization, passivation, and glow-discharge nitrogen implantation are used for further strengthening (1).
This group of stainless steels is not magnetic and has good resistance to corrosion due to the 14% to 15% nickel content. The low amount of carbon content and the chromium and molybdenum further improve corrosion resistance. A layer of oxidation provides protection to some forms of corrosion. This group of stainless steel still may corrode within the body under circumstances such as areas that lack oxygen (screw-plate interface or in high-stress areas).
COBALT-CHROMIUM-BASED ALLOYS
There are two types of cobalt-chromium alloys: castable CoCrMo alloy and CoNiCrMo alloy. The castable CoCrMo alloy is used in manufacturing artificial joints. This surface can be highly polished and is most compatible against an opposing surface of polyethylene. This metal however does not have the best bone integration properties. Thus, some manufacturers will coat cobalt-chromium implants with titanium on the surfaces that are in contact with bone.
TITANIUM
Titanium was first used in the 1930s for the fabrication of implants. Titanium has the lowest specific gravity of metals used for designing implants and hence has a very light weight (4.5 g/cm compared with 7.9 g/cm2 for 316L stainless steel). Four grades of titanium are used for implant fabrication. The amounts of oxygen, iron, and nitrogen distinguish the four groups (Table 2.2). The titanium alloy Ti6A14V is the alloy that is primarily used for the design and construction of the implant.
Applying alloying elements to titanium broadens the range of mechanical properties. The two main alloying agents are aluminum and vanadium. The higher impurity content increases the strength while having an opposite effect on ductility (2). The strength of titanium varies from a value much lower than 316L stainless steel of the CoCr alloys to a value about equal to the annealed 316L stainless steel and casted CoCrMo alloy. However, when compared by the specific strength (strength per density), the titanium alloys exceeds any other implant material (3). Titanium is relatively inert and has good integration into the surrounding bone. It can integrate into bone and essentially form a proteoglycan layer that aids adherence between the implant and the adjacent tissue (4). Osseointegration is defined as direct contact between viable remodeled bone and an implant, without intervening soft tissue. The surface roughness of titanium alloys has a significant effect on the bone apposition to the implant
and on the bone interfacial pullout strength. Highest levels of osteoblast cell attachment are obtained with rough sand blast surfaces, where cells are differentiated more than those on smooth surfaces (5). Chemical changes of the titanium surface following heat treatment are thought to form a TiO2 hydrogel layer on top of the TiO2 layer. The TiO2 hydrogel layer may induce apatite crystal formation (6). It also possesses a modulus of elasticity that is half of stainless steel, which offers an advantage for load transfer into bone. The disadvantage of titanium is its poor shear strength that makes it less desirable compared with 316L stainless steel for bone screws and plates.
and on the bone interfacial pullout strength. Highest levels of osteoblast cell attachment are obtained with rough sand blast surfaces, where cells are differentiated more than those on smooth surfaces (5). Chemical changes of the titanium surface following heat treatment are thought to form a TiO2 hydrogel layer on top of the TiO2 layer. The TiO2 hydrogel layer may induce apatite crystal formation (6). It also possesses a modulus of elasticity that is half of stainless steel, which offers an advantage for load transfer into bone. The disadvantage of titanium is its poor shear strength that makes it less desirable compared with 316L stainless steel for bone screws and plates.
TABLE 2.1 316L Stainless Steel Composition | ||||||||||||||||||||
---|---|---|---|---|---|---|---|---|---|---|---|---|---|---|---|---|---|---|---|---|
|
TABLE 2.2 Chemical Composition of Titanium and Its Alloy | ||||||||||||||||||||||||||||||||||||||||||||||||||||||||||
---|---|---|---|---|---|---|---|---|---|---|---|---|---|---|---|---|---|---|---|---|---|---|---|---|---|---|---|---|---|---|---|---|---|---|---|---|---|---|---|---|---|---|---|---|---|---|---|---|---|---|---|---|---|---|---|---|---|---|
|
CORROSION OF METALLIC IMPLANTS
Corrosion is defined as the chemical reaction of a metal with its environment, resulting in continued degradation (7). All implanted metallic hardware will have some type of reaction with the surrounding material. The tissue fluid within the human body presents a very aggressive environment for corrosion. The surface-tissue interaction is dynamic rather than static and will develop into new stages as time passes, especially after initial implantation. During the initial few seconds after implantation, there will be only water, dissolved ions, and free biomolecules in the closest proximity of the surface, but no cells. The composition of biofluid will then change continuously as inflammatory and healing processes proceed, which in turn also probably causes changes in the absorbed layer of biomolecules on the implant surface until equilibrium sets in. Eventually cells and tissues will approach the surface, and depending on the nature of the adsorbed layer, they will respond in specific ways that will further modify the adsorbed molecules (8,9,10 and 11).
Corrosion in metals is divided into a variety of interactions. Galvanic corrosion occurs when two dissimilar metals are present in the same environment. The one that is most negative in the galvanic series will become the anode and site of galvanic corrosion. This corrosion is more rapid than single-metal corrosion; therefore, implantation of widely dissimilar metals is to be avoided. Fretting corrosion (also known as crevice corrosion) is a mechanical rubbing of one part on another, which disrupts the passivation layer, in an area of low oxygen, resulting in localized corrosion (Fig. 2.2). Crevice corrosion can occur between the contact area between a screw and plate. Stainless steel is vulnerable to pitting. Pitting is a form of localized corrosion in which pits form on the metal surface. Mechanical stress can also accelerate the corrosion process. As mechanical stress is repeated, fatigue stress corrosion takes place. Fretting corrosion certainly can occur in this situation as well (12,13). Frictional corrosion is most significant whereby debris is generated from contact abrasion and micromotion of the implants at the osseous surface. Metal particles accumulate in adjacent soft tissue. The effects of tissue depend on the particle size, the concentration of duration and exposure, and the surface characteristics of the implant (2).
Clinically corrosion of implants can cause localized pain and swelling over the implant. Cracking or flaking of the implant
is occasionally visible on radiographs. Surgically, the soft tissue that surrounds the implant can have a black or gray discoloration with the evidence of flaking around the adjacent soft tissue (Fig. 2.3). Metallosis is the release of corrosion debris into the surrounding soft tissue. Titanium debris can be found within the connective tissue free of macrophage invasion, whereas stainless steel is incorporated into macrophages and giant cell bodies. Titanium dioxide, the ion released, is believed to be of low tissue toxicity compared with the stainless steel debris (14). Black metallic wear debris secondary to titanium implants has been implicated in titanium cyst formation in bone as well as in soft tissue pigmentation visible on the removal of the implant. (14,15) (Fig. 2.4)
is occasionally visible on radiographs. Surgically, the soft tissue that surrounds the implant can have a black or gray discoloration with the evidence of flaking around the adjacent soft tissue (Fig. 2.3). Metallosis is the release of corrosion debris into the surrounding soft tissue. Titanium debris can be found within the connective tissue free of macrophage invasion, whereas stainless steel is incorporated into macrophages and giant cell bodies. Titanium dioxide, the ion released, is believed to be of low tissue toxicity compared with the stainless steel debris (14). Black metallic wear debris secondary to titanium implants has been implicated in titanium cyst formation in bone as well as in soft tissue pigmentation visible on the removal of the implant. (14,15) (Fig. 2.4)
![]() Figure 2.2 Fatigue fracture of chromium-cobalt. A is 50× magnification; B is 150× magnification. (Courtesy of Wright Medical Technology, Inc.) |
Titanium implants have very little corrosion due to the passivation layer. This passivation layer occurs by forming a solid oxide layer. Titanium offers superior corrosion resistance, but it is not as stiff or strong as stainless steel or cobalt-chromium alloys. Cobalt-chromium implants are also passive in the human body, and they do not exhibit pitting corrosion. Stainless steel 316L contains molybdenum, which confers greater resistance to pitting but is vulnerable to both pitting and crevice corrosion.
PYROLYTIC CARBON
Pyrolytic carbon is an attractive material in the development of joint prosthesis due to its excellent biomechanical properties (Fig. 2.5). A “ceramic-like isotropic coating over a graphite substrate” (16), it is formed by the pyrolysis of a hydrocarbon gas and has the physical and mechanical properties between graphite and diamond (17,18). The characteristics (anisotropy, density, crystallite size, and structure) of the deposited carbon should be highly controlled during the deposition process since the formation of growth features associated with uneven crystallization can result in a weaker material (19).
One of the characteristics that make pyrolytic carbons an attractive implant material is the similar elastic modulus to cortical bone with a higher durability and wear resistance. This minimizes the amount of stress at the bone-implant interface
as well as wear debris and inflammatory reaction (20,21 and 22). The reported wear rate is less than 10% of that of ultrahigh-molecular-weight polyethylene (UHMWPE), and there is no evidence of fatigue fracture after 8 to 10 million load cycles (16,23). All of these characteristics potentially make the pyrolytic carbons a better choice for active, younger patients.
as well as wear debris and inflammatory reaction (20,21 and 22). The reported wear rate is less than 10% of that of ultrahigh-molecular-weight polyethylene (UHMWPE), and there is no evidence of fatigue fracture after 8 to 10 million load cycles (16,23). All of these characteristics potentially make the pyrolytic carbons a better choice for active, younger patients.
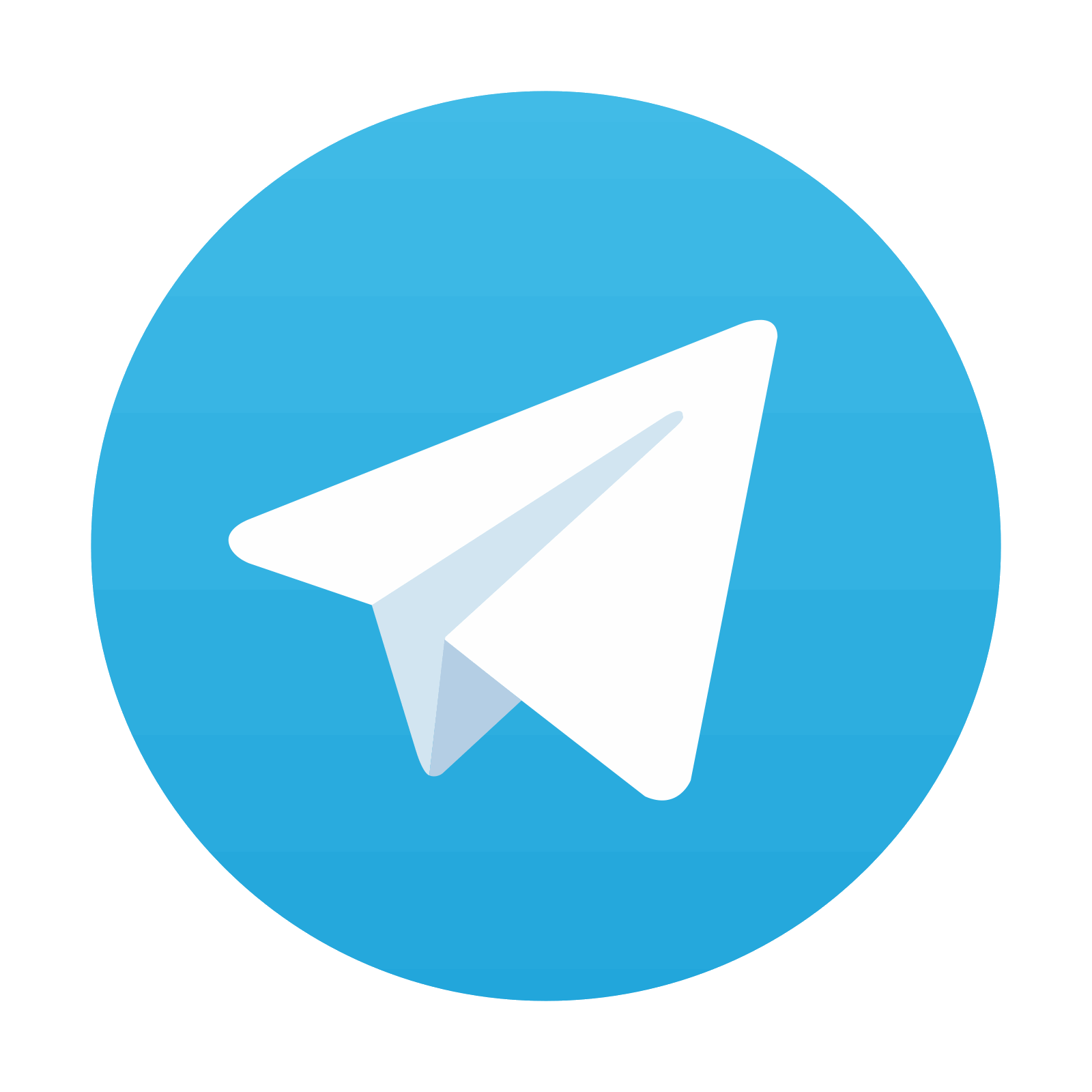
Stay updated, free articles. Join our Telegram channel
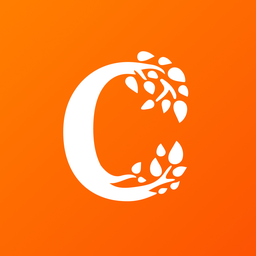
Full access? Get Clinical Tree
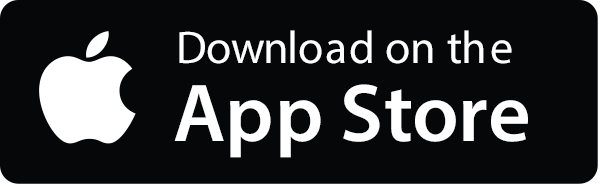
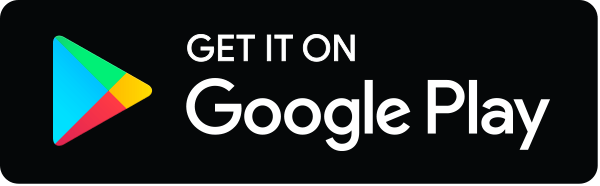