Articular Cartilage and Labrum: Composition, Function, and Disease
Linda J. Sandell
Ken Takebe
Shingo Hashimoto
Corey S. Gill
Articular Cartilage
Composition
Normal articular cartilage consists of an extensive, hydrated extracellular matrix (ECM) that is synthesized and maintained by a sparse population of specialized cells, the chondrocytes. In the adult human, chondrocytes may occupy as little as 2% of the total volume of the hip articular cartilage (1,2). The surface layer of articular cartilage is in direct contact with articular synovial fluid and is not covered by a perichondrium (3).
In the adult knee, the macroscopic structure of articular cartilage is maintained by resident chondrocytes, which compose only about 5% of the wet weight of articular cartilage and <10% of the cartilage tissue volume (3,4). The mean thickness of human knee articular cartilage is 1.36 to 2.48 mm, with the thickest cartilage located on the patella (5). On the other hand, in the hip joint, the acetabular articular cartilage is generally thickest superolaterally, averaging 1.83 mm compared with an average thickness of 1.26 mm in other acetabular areas (6). The femoral head articular cartilage is generally thickest anteromedially, averaging 1.84 mm compared with an average thickness of 1.40 mm in other femoral head areas (6). Articular cartilage of the hip is structurally and functionally divided into four zones: the superficial zone, the middle zone, the deep zone, and the calcified zone (7,8,9,10) (Fig. 4.1). The superficial zone forms a smooth gliding surface between the femoral head and acetabulum (1,8,11). The superficial zone of articular cartilage is composed of tightly woven sheets of collagen fibers oriented parallel to the articular surface (1,4,10,11). This zone makes up approximately 10% to 20% of articular cartilage thickness (8). Proteoglycan concentration is lower in the superficial zone compared with other zones of articular cartilage (10,11), whereas fibronectin and water concentrations are highest in this zone (10). Similar to the orientation of collagen fibrils, chondrocytes in the superficial zone are flattened and aligned parallel to the articular surface (4,10,12). The dense mat of collagen fibrils lying parallel to the joint surface in the superficial zone impart this layer of cartilage with high tensile stiffness and strength and probably acts to resist compressive forces generated during normal joint function (10).
The intermediate zone of articular cartilage is the thickest zone, encompassing 40% to 60% of the articular cartilage volume (8). In this region, the collagen fibers are thick, less organized, and are typically in an oblique orientation to the articular surface (4,8,11,12). This level has a high proteoglycan content but a lower concentration of water and collagen than that of the superficial zone (10,11). Chondrocyte morphology in the intermediate zone is more rounded than the flattened chondrocytes of the superficial zone (8).
In the deep zone of articular cartilage, chondrocytes and collagen fibers are oriented in vertical columns perpendicular to the articular cartilage surface (4,8). This zone has the highest concentration of proteoglycan and the lowest concentration of water (8,10,11). Cellular density of articular cartilage is the highest in the superficial layer and gradually decreases through the intermediate and deep zones. The cellular density of the deep zone is approximately one-third of the density of the superficial zone (4,13). In contrast to cellular density, both cell volume and the proportion of proteoglycan relative to collagen increase from the superficial zone to the deep zone (13).
The partly calcified cartilage layer provides a buffer with intermediate mechanical properties between those of the uncalcified cartilage and the underlying subchondral bone (12,13). The zone of calcified cartilage is a small layer, consisting of radially oriented collagen fibers embedded in a calcified matrix (11). The chondrocytes in this calcified zone usually express the hypertrophic phenotype similar to the growth plate (12). Here, collagen fibers traverse into the tidemark, which represents a relative change from the deep zone to the zone of calcified cartilage (11). The number of tidemarks increases with age as the tissue is remodeled (9). These tidemarks serve a physiologic barrier between subchondral bone and articular cartilage, with no evidence to suggest that nutrients from the underlying bone traverse the tidemark (10).
From a developmental standpoint, chondrocytes are derived from mesenchymal cells, which differentiate during skeletal morphogenesis and development to form chondrocytes (9). Chondrocytes contain the organelles such as the endoplasmic
reticulum and Golgi apparatus necessary for matrix synthesis (10). The cells also contain structures necessary for the maintenance of matrix, such as intracytoplasmic filaments, lipids, glycogen, and secretory vesicles (10). Chondrocytes surround themselves with ECM and unlike osteocytes do not form cell-to-cell contacts (10). Despite their lack of direct cell-to-cell contacts, chondrocytes are still able to orchestrate the balance between matrix synthesis and breakdown that facilitates normal cartilage homeostasis. Chondrocyte metabolism is influenced by multiple factors, including composition of the surrounding matrix, mechanical load, hormones, local growth factors, cytokines, aging, and injury (4). This coordinated metabolism enables chondrocytes to efficiently coordinate their primary function, producing and maintaining the ECM that will be compressible in articular cartilage (3).
reticulum and Golgi apparatus necessary for matrix synthesis (10). The cells also contain structures necessary for the maintenance of matrix, such as intracytoplasmic filaments, lipids, glycogen, and secretory vesicles (10). Chondrocytes surround themselves with ECM and unlike osteocytes do not form cell-to-cell contacts (10). Despite their lack of direct cell-to-cell contacts, chondrocytes are still able to orchestrate the balance between matrix synthesis and breakdown that facilitates normal cartilage homeostasis. Chondrocyte metabolism is influenced by multiple factors, including composition of the surrounding matrix, mechanical load, hormones, local growth factors, cytokines, aging, and injury (4). This coordinated metabolism enables chondrocytes to efficiently coordinate their primary function, producing and maintaining the ECM that will be compressible in articular cartilage (3).
The composition of articular cartilage is unique compared to other tissues for several reasons. Articular cartilage has no direct nervous system supply. Consequently, it is not the articular cartilage itself that sends pain signals to the body in disease states such as osteoarthritis (OA) and femoroacetabular impingement (FAI), but rather inflammation or damage to surrounding tissues such as the synovium and bone. Articular cartilage is unlikely to display significant immune responses (cellular or humoral) in response to antigens since both monocytes and immunoglobulins tend to be excluded from the tissue by steric exclusion (9). In the calcified cartilage zone, hypertrophic chondrocytes are unique in that they synthesize type X collagen and can calcify the ECM (12). Unlike in bone formation, this calcified matrix is not resorbed fully in development and ordinarily resists vascular invasion (12). Ruiz-Romero et al. (14) demonstrated that there are 93 different proteins in normal articular chondrocytes, and these proteins are primarily involved in cell organization (26%), energy production (16%), protein fate (14%), metabolism (12%), and cell stress (12%).
Although chondrocytes are the primary cellular component of articular cartilage, the ECM produced by chondrocytes imparts many of the unique functions and properties of cartilage. The ECM is a hyperhydrated tissue, with values for water ranging from 60% to almost 80% of the total wet weight. The remaining 20% to 30% of the wet weight of the tissue is principally accounted for by two macromolecular proteins: type II collagen, which composes up to 60% of the dry weight, and the large highly negatively charged
proteoglycan, aggrecan, which accounts for a large part of the remainder (3,9). Several other classes of molecules, including lipids, phospholipids, proteins, and glycoproteins, make up the remaining portion of the ECM (9). Although each of these factors plays a role in cartilage homeostasis, type II collagen is unarguably the major structural protein of ECM. Type II collagen is the major fibrillar collagen of articular cartilage, and constitutes 90% to 95% of total collagen and 10% of the wet weight of articular cartilage (4). Collagen fibers in cartilage are generally thinner than those seen in tendon or bone, and this may in part be a function of their interaction with the relatively large amount of proteoglycan in this tissue, interaction with other collagens and small proteoglycans and intrinsic differences in collagen amino acid sequence (9). The fibers in articular cartilage vary in width from 10 to 100 nm, although their width may increase with age and disease (9). Type II collagen forms a highly cross-linked and interconnected network of collagen fibrils (4) that contribute to the shear and tensile properties of the tissue (8). Like all collagens, type II collagen contains a characteristic triple helix structure (8).
proteoglycan, aggrecan, which accounts for a large part of the remainder (3,9). Several other classes of molecules, including lipids, phospholipids, proteins, and glycoproteins, make up the remaining portion of the ECM (9). Although each of these factors plays a role in cartilage homeostasis, type II collagen is unarguably the major structural protein of ECM. Type II collagen is the major fibrillar collagen of articular cartilage, and constitutes 90% to 95% of total collagen and 10% of the wet weight of articular cartilage (4). Collagen fibers in cartilage are generally thinner than those seen in tendon or bone, and this may in part be a function of their interaction with the relatively large amount of proteoglycan in this tissue, interaction with other collagens and small proteoglycans and intrinsic differences in collagen amino acid sequence (9). The fibers in articular cartilage vary in width from 10 to 100 nm, although their width may increase with age and disease (9). Type II collagen forms a highly cross-linked and interconnected network of collagen fibrils (4) that contribute to the shear and tensile properties of the tissue (8). Like all collagens, type II collagen contains a characteristic triple helix structure (8).
Type IX and XI collagen are the most abundant minor collagen types within articular cartilage and are present in roughly equal amounts (approximately 1:10 compared with type II collagen) (4). Type IX is a short fibrillar collagen that contains a proteoglycan moiety. It forms cross-links with type II collagen along the surface of collagen fibrils and integrates with proteoglycan aggregates in the ECM (4). On the other hand, type XI collagen forms fibrils, and its main function seems to be as a regulator of the fibril diameter of type II collagen, with which it forms copolymers (4).
Aggrecan is a highly glycosylated protein produced primarily in chondrocytes (4). A single aggrecan molecule consists of protein core and numerous highly charged glycosaminoglycan side chains (15). In normal articular cartilage, many aggrecan molecules bind to a chain of hyaluronan, and this interaction is stabilized by separate link protein (1). Aggrecan molecules fill most of the interfibrillar space of the cartilage matrix (10). They contribute about 90% of the total cartilage matrix proteoglycan mass, whereas large nonaggregating proteoglycans contribute 10% or less and small nonaggregating proteoglycans contribute about 3% (10). The proteoglycans are negatively charged due to the presence of carboxyl and sulfate groups on the glycosaminoglycans, and so confer a net negative charge on the cartilage ECM (15). As a result, cartilage is highly hydrophilic, with a tendency to imbibe fluid, or swell, to maintain mechanochemical equilibrium (15).
The organization of the ECM and the distribution of zones are slightly different in immature versus mature cartilage. In young individuals, the layer of articular cartilage is generally much thicker and unstratified, with chondrocytes being distributed in a more random, isotropic pattern. As the tissue matures, there is a much higher degree of anisotropy with cells and matrix being arranged into the clearly defined zones (3).
Water constitutes approximately 75% of the weight of articular cartilage. The water content is lower in the superficial layers; approximately 65% is found in the deeper layers (4). Articular cartilage resists compressive forces because of its high hydrostatic pressure (4). During the early phases of OA, water content may increase to over 90% before disintegration of the tissue occurs (9). Inorganic salts, such as sodium, calcium, chloride, and potassium, are dissolved in the water (9). With compression, there is an egress of water from the cartilage, which provides a thin layer of liquid that further reduces friction and facilitates the gliding of opposing articular surfaces (4). One of the earliest changes in OA is loss of the integrity and interconnectivity of the collagen matrix. The increased osmotic pressure causes swelling. Subsequent loss of proteoglycans leads to loss of osmolality and further compromise of the mechanical properties (4).
Because articular cartilage is avascular, chondrocytes derive both oxygen and nutrition from the synovial fluid by simple diffusion (4). The oxygen tension in cartilage may be as low as 1% to 3%, compared with 21% atmosphere (4). The energy requirements of chondrocytes are met primarily through glycolysis, whereby glucose is metabolized under anaerobic conditions into lactate (4).
Macroscopically, the hip acetabular articular cartilage surface is horseshoe shaped with a central recessed area devoid of articular cartilage. This area is called the pulvinar and does not directly articulate with the femoral head (16). The femoral head is completely covered with articular cartilage with the exception of the attachment of the ligamentum teres (16). The hip joint cartilage is thinner in comparison to knee cartilage with the maximum thickness ventrocranially at the acetabulum and ventrolaterally on the femoral head (16).
Biomechanics (Function)
The primary function of the articular cartilage of the hip joint is to provide a smooth, congruent gliding surface between the acetabulum and the femoral head. This function enables painless and efficient movement of the hip joint in flexion/extension, abduction/adduction, and rotation. Efficient and wear-resistant motion of the hip joint is critical for almost all activities of daily living such as ambulation and sitting, as well as recreational activities and athletics. Critical to the function of articular cartilage is the intimate relationship between the collagen matrix and aggregating proteoglycans (4). Articular cartilage serves as a low-friction, wear-resistant surface for load support, load transfer, and motion between the bones of the diarthrodial hip joint (2). Joint loading and motion are required to maintain normal adult articular cartilage composition, structure, and mechanical properties (9). The type, intensity, and frequency of loading necessary to maintain normal articular cartilage vary over a broad range (9). When the intensity or frequency of loading exceeds or falls below these necessary levels, the balance between synthesis and degradation processes will be altered, and changes in the composition and microstructure of cartilage follow (9).
Articular cartilage is subjected to a wide range of static and dynamic mechanical loads (10). Under normal physiologic conditions, in vivo loading can result in peak dynamic mechanical stresses on cartilage as high as 15 to 20 MPa during activities such as stair climbing (10). Because collagen and proteoglycan form a fiber-reinforced composite material, the collagen network provides shear stiffness and strength to the tissue, enabling it to withstand these high stresses (17). Under physiologic conditions, collagen metabolism is slow, and fibrils have a half-life of years (4). However, in disease states, turnover can increase markedly and can exceed the ability of chondrocytes to produce a
well-organized replacement matrix (4). The ability of cartilage to withstand physiologic compressive, tensile, and shear forces depends on the composition and structural integrity of its ECM (10). In turn, the maintenance of a functionally intact matrix requires chondrocyte-mediated synthesis, assembly, and degradation of proteoglycans, in addition to other matrix molecule proteins (10).
well-organized replacement matrix (4). The ability of cartilage to withstand physiologic compressive, tensile, and shear forces depends on the composition and structural integrity of its ECM (10). In turn, the maintenance of a functionally intact matrix requires chondrocyte-mediated synthesis, assembly, and degradation of proteoglycans, in addition to other matrix molecule proteins (10).
Measurements have revealed that the equilibrium compressive modulus of adult articular cartilage is in the order of approximately 0.5 to 1 MPa, the shear modulus about 0.25 MPa, and the tensile modulus about 10 to 50 MPa (10). Several factors have been shown to alter these material properties. Kempson (18) showed that tensile properties of the network of collagen fibrils of the femoral head deteriorate considerably with increasing age. Other studies have shown that joint loading can induce a wide range of metabolic responses in cartilage. Immobilization can cause decreases in matrix synthesis and content and a resultant softening of the tissue (10). In contrast, aggrecan concentration is higher in areas of loaded cartilage and appears to restore the cartilage structure (10).
In evaluating the structural properties of hip articular cartilage, Athanasiou (17) found that the aggregate modulus is 1.207 MPa, Poisson ratio is 0.045, permeability is 0.895 × 10-15 m4/N · s, and thickness is 1.34 mm. For cartilage of the human knee, the corresponding values are 0.604 MPa, 0.060, 1.446 × 10-15 m4/N · s, and 2.631 mm. Thus, cartilage in the human hip joints is twice as stiff, less permeable, and half as thick compared with cartilage in the knee (6).
Disease
Biomechanically, the hip joint links the lower extremity to the torso, and experiences high amounts of stress during activities of daily living and recreational activities. Weight-bearing stresses on the hip during walking can be greater than five times an individual’s body weight (19). If articular cartilage of the hip is damaged by trauma, disease, or aging, the end result is OA. OA of the hip joint can be classified into two subgroups: primary OA and secondary OA. Primary OA is idiopathic and occurs with higher frequencies in older adults, whereas secondary OA has a defined etiology such as developmental dysplasia of the hip (DDH), Perthes disease, trauma, or FAI that lead to the development of degenerative changes. Both primary and secondary OA show articular cartilage degeneration, erosion, cartilage loss, and subchondral bone sclerosis (20). We have recently reviewed the potential genetic contributions to hip joint structure and OA (21). With improved understanding of the structural etiology of hip OA, primary OA is thought to be relatively uncommon (22).
DDH, previously known as congenital dislocation of the hip joint, often results in hip instability characterized by insufficient anterolateral femoral head coverage and superolateral inclination of the acetabular articular surface (23) (Fig. 4.2). Anterolateral acetabular rim overload, instability, and excessive shear stresses lead to early joint degeneration (24). OA due to DDH most often becomes symptomatic in middle-aged adults and then progressively worsens to end-stage arthritis and subsequent hip arthroplasty (25). Since the natural history of untreated DDH often leads to significant morbidity, many surgeons recommend surgical interventions in childhood or adolescence to promote development of the acetabulum and/or to increase stability of the hip joint. A variety of techniques have been developed to accomplish these goals, such as open and closed hip reductions, as well as a number of femoral and pelvic osteotomies (26,27,28,29,30,31,32,33).
Perthes disease is an idiopathic osteonecrosis of the epiphysis of proximal femur in children and was first described independently by Legg, Calvé, and Perthes in 1909 and 1910 (34). The cause of this disease is not clearly identified, but disordered chondrogenesis as well as minor trauma, thrombosis, and abnormal blood supply have been implicated as possibilities (35,36,37
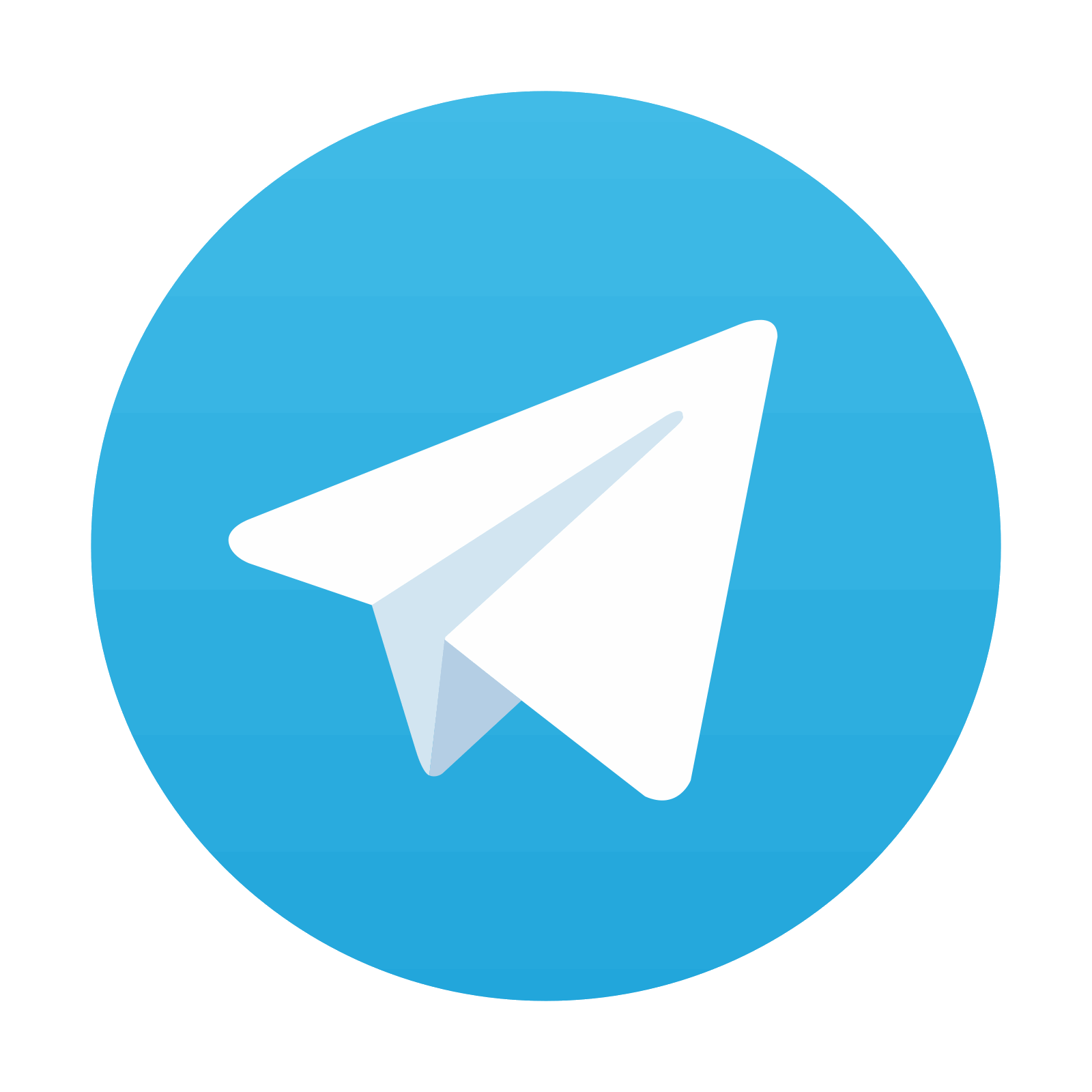
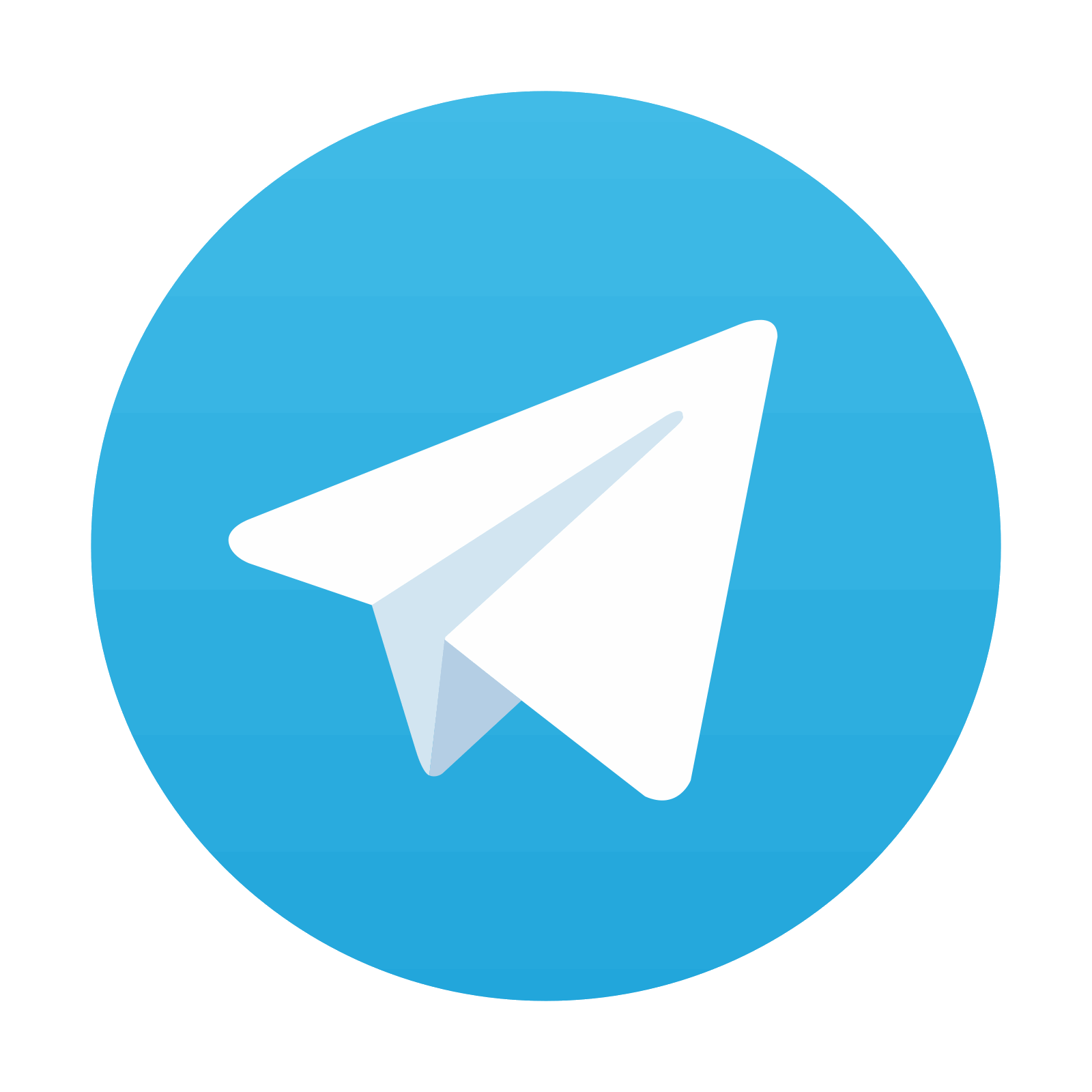
Stay updated, free articles. Join our Telegram channel
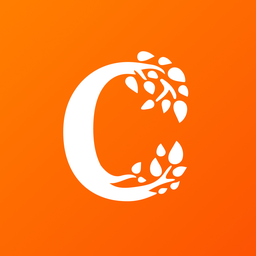
Full access? Get Clinical Tree
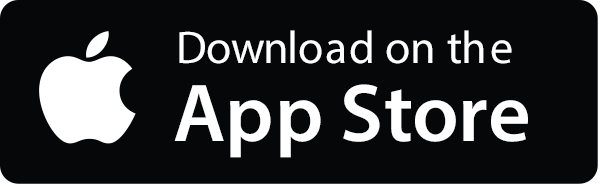
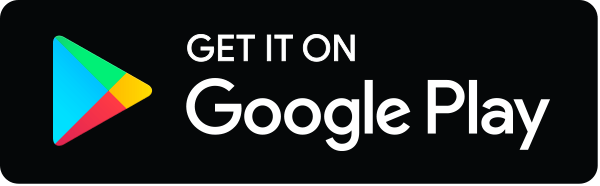
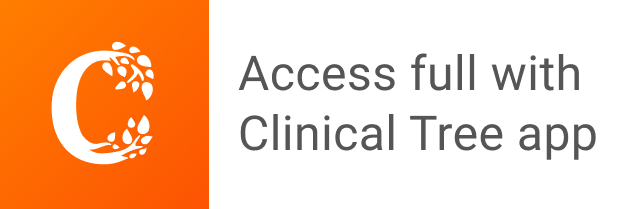