Fig. 25.1
The meniscus is a semilunar fibrocartilaginous structure
Traumatic injury and/or degenerative changes disrupt the mechanical function of these tissues. These changes can lead to the early onset and accelerate the development of osteoarthritis. The current standard treatment is meniscectomy, or resection of the damaged portion of the meniscus, a procedure that fails to regenerate normal knee mechanics or prevent the initiation of osteoarthritic cascades.
Microstructure and Composition
About 70–75 % of the wet weight of the meniscus consists of water [2, 5–7]. The dry weight consists of 60–70 % collagen, 1 % proteoglycans, and 8–13 % non-collagenous proteins (such as elastin). Collagens are primarily type I (90 %) with smaller amounts of II, III, V, and VI [6, 8]. The collagen fibres are mainly oriented circumferentially, with some radially oriented fibres (Fig. 25.2). This may be related to the mechanical forces that act on the menisci.
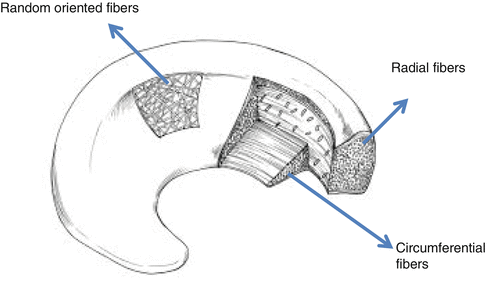
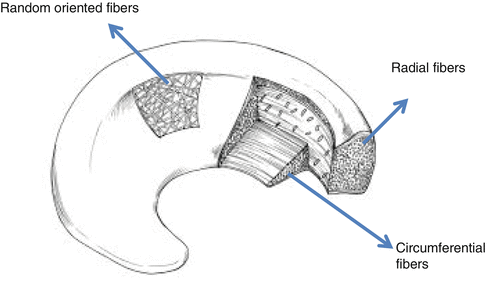
Fig. 25.2
Schematic diagram of the ultrastructure of collagen fibers of the meniscus
Fibrochondrocytes are the predominant cell type in the meniscus. They produce collagen and its extracellular matrix [8].
Along the inner avascular zone, cells are morphologically similar to articular chondrocytes but on the periphery, cells are more similar to fibroblasts. Arnoczky et al. demonstrated that the outer 10–30 % of the medial meniscus and 10–25 % of the lateral meniscus is vascular [9].
The medial and lateral geniculate arteries form a perimeniscal capillary plexus that supplies the outer surface of the menisci. The menisci have intrinsic innervation. Innervation is most abundant on the periphery and the anterior and posterior horns [10]. The meniscus does have an inherent ability to heal itself, but this is limited to the vascularized periphery [11].
Proprioception is believed that obtained from free nerve endings and they are activated on the anterior and posterior horns during flexion and extension of the knee [12, 13].
The unique architecture of the meniscus consists of circumferentially oriented collagen fibers interspersed with radial collagen fibers [2].
Biomechanics of the Menisci
The mechanical properties of this tissue are highly anisotropic (different in opposing directions), and strongly dependent on the prevailing fiber direction [14, 33]. This can be seen in the tensile stress–strain response of samples oriented in the circumferential direction as compared to those oriented in the radial direction. Circumferential samples show a pronounced “toe” region common to fiber-reinforced tissues, and a higher linear modulus thereafter. Radial samples are relatively linear in their stress–strain response, with a much lower modulus. The tensile properties of the meniscus range from 48 to 259 MPa in the circumferential direction and 3–70 MPa in the radial direction, depending on anatomic location and species [2, 14, 15, 33]. The compressive properties of the meniscus are low relative to articular cartilage (50–400 kPa, about one-half) [16]. The meniscus, less stiff in compression, is also much less permeable than articular cartilage [2], suggesting that the tissue is optimized to enhance congruency, load distribution, and shock absorption across the joint [33].
Load distribution over an incongruent joint surface is redistributed by the menisci by maintaining maximal congruency. The functionality of the menisci and their role in load transmission across the knee has been discussed by many authors [2, 17].
The main functions of the meniscus are to increase joint congruency, transmit and distribute compressive load between the femur and the tibial plateau, stabilize the joint, and improve articular cartilage nutrition and lubrication [7]. These functions are achieved by the unique load transfer that occurs between the (more hyaline) inner region and the (more fibrous) outer region of the meniscus. When the joint is loaded vertically, axial loads from the femoral condyles are redirected laterally .Lateral extrusion of the meniscus is resisted by the osseous anchorage of the anterior and posterior horns [33], generating hoop stresses within the dense network of circumferentially oriented collagen fibers [18]. Normally, the menisci transmit 50–100 % of the loads in the knee (multiples of body weight) [19], with tensile deformations limited to 2–6 % [20, 21].
The main role of the menisci in the knee joint is load bearing. When bearing load, the knee joint is subjected to compression. The compressive force through the joint is distributed over an articulating contact area resulting in contact stresses (contact pressure). The menisci optimize the way that load is transferred across the knee joint by increasing the congruency of the articulation. The areas of contact increase and, as a result, the contact pressure on the articulating surfaces decreases. As the femoral condyles bear down onto the menisci, the meniscal wedged cross section causes the menisci to extrude radially out of the joint; this causes their circumference to increase. Each meniscus attaches mainly to the tibia by anterior and posterior insertional ligaments. Thus, the bulk tissue resists radial displacement by developing circumferential tension (hoop stresses) (Fig. 25.3); this is due to the stiffness of the meniscal tissue, with the predominantly circumferential orientation of the collagen fibres.
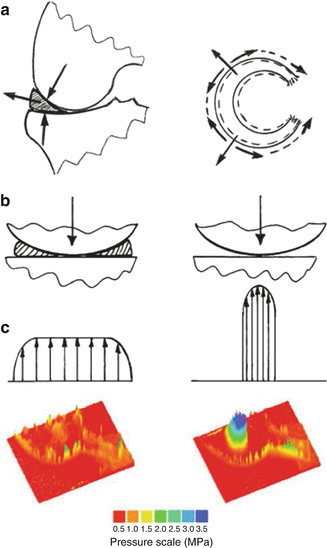
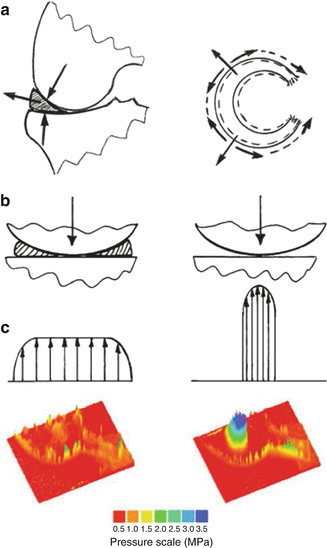
Fig. 25.3
(a) Load transfer through the knee joint. The menisci extrude under axial joint load. (b) Contact areas decrease and contact stresses increase following meniscectomy. (c) Contact stress distribution (in MPa) in the tibial cartilage of the intact and meniscectomized joint
The lateral meniscus is more mobile than the medial meniscus because it is not as tightly attached to the capsule as the medial meniscus. Also, the concave medial tibial plateau does not allow the posterior aspect of the medial meniscus to displace off the joint posteriorly in deep flexion; however, the convex posterior aspect of the lateral tibial plateau allows the lateral meniscus to displace posteriorly (go “downhill”) in deep flexion. These key factors may explain the increased frequency of medial meniscal tears compared to lateral meniscal tears, at a ratio of 2:1 [22].
The variable effect and frequency of the various types of meniscal tear may depend on the resistance of the menisci to compressive loads by increasing their circumference and developing hoop stresses.
The menisci distribute contact forces over the articular surfaces effectively by increasing the contact surface of the joint. The menisci maintain maximal congruency by redistribution of the load, over an incongruent joint surface. The functionality of the menisci and their role in load transmission across the knee has been discussed by many authors [2, 17].
Havitcioglu et al. investigated the biomechanical properties of meniscus under pull-out and compression forces (Fig. 25.4) [23]. In compression–tensile tests, statistically significant difference was observed between force and displacement values [(p = 0.037) and (p = 0.045), respectively] (Tables 25.1 and 25.2).
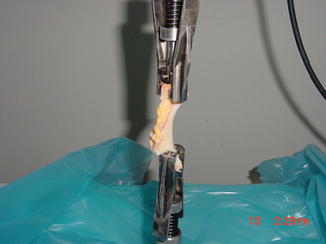
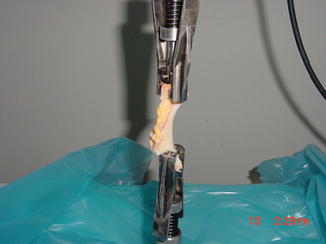
Fig. 25.4
Photograph of meniscus during the tensile test
Table 25.1
Force and displacement values in compression tests
Force (N) | Displacement (mm) |
---|---|
4494.06 | 9.83 |
4498.75 | 7.21 |
4501.20 | 3.99 |
4495.94 | 8.43 |
4497.34 | 6.67 |
Table 25.2
Force and displacement values in pull-out tests
Force (N) | Displacement (mm) |
---|---|
17.50 | 6.60 |
24.06 | 6.04 |
21.40 | 5.09 |
55.93 | 16.6 |
105.05 | 14.37 |
29.21 | 11.10 |
22.18 | 3.87 |
29.21 | 4.08 |
46.56 | 9.34 |
Knowledge of the detail properties of the meniscus and cell culture biomechanics will help the artificial meniscus basic studies. Biomechanical knowledge of the properties of natural meniscus are light is keep for future studies.
Finite Element
With respect to meniscus biomechanics and its possible degeneration, finite element simulations help to understand the stress distribution in the human knee joint and prevent joint injuries and pathological degeneration of articular joints. Three-dimensional finite element models can also be used to estimate the consequences of surgical treatments such as total or partial meniscectomies. [24] considered the effect of meniscectomies on the human knee joint using the finite element method.
Some study was to develop a three-dimensional finite element model of the human tibio-femoral joint including the femur, tibia, cartilage layers, menisci, and main ligaments to estimate the contact areas and pressure distributions between menisci and articular cartilage and the stress distribution in the articular cartilage to investigate the effect of meniscal tears and meniscectomies on these variables. This could help to explain the cartilage degeneration after a total or partial meniscectomy [25, 26].
A 3D model was developed of the tibio-femoral human knee joint including femur, tibia, cartilage layers, menisci, and joint ligaments and analyzed under axial compression forces. It allows a better understanding of the role of the menisci in the transmission of forces across the knee and to investigate the effect of meniscal tears and meniscectomies on the biomechanics of human knee joint [27].
Degeneration and Functional Biomechanics of the Degenerative Meniscus
Normal menisci are semilunar, fibrocartilage structures (Fig. 25.1). Deviations from this can occur due to disease, degeneration, traumatic injury, or abnormal development. Once torn, or damaged, the chondroprotective ability of the tissue is disrupted and the cascade toward osteoarthritis initiated [28]. Osteoarthritis leads to degenerative changes in the menisci with the surrounding cartilage, and this is related to 75 % of all meniscal tears and extrusions [29, 30].
Several authors suggested that damage in cartilage starts at its surface and extends through the thickness. This can be explained by a maximal shear stress criterion [31]. Moreover, loss of meniscal tissue frequently leads to long-term degenerative joint changes, articular cartilage degeneration, and osteoarthritis [32, 33].
Most authors agree that total meniscectomy leads to progressive articular wear after a few years. This is a fact that the global biomechanics of the knee is altered and the articular instability increases; so this state can be resulted a progressive and degenerative arthrosic pathology [17, 34].
With consider to meniscus biomechanics and its possible degeneration, finite element simulations help to understand the stress distribution in the human knee joint, so this will help us to prevent joint injuries and pathological degeneration of articular joints. Three-dimensional finite element models can also be used to predict the results of surgical treatments such as total or partial meniscectomies.
Wilson et al. considered the effect of meniscectomies on the human knee joint using the finite element method. They determined the stress and strain distributions and fluid velocities in the articular cartilage before and after meniscectomy, but only used an axisymmetric model [24]. In another study, Pena et al. investigated the effect of meniscal tears and meniscectomies on the human knee joint. They use a 3D finite element model that included the femur, tibia, cartilage layers, menisci, and ligaments. Three different situations were compared: a healthy tibio-femoral joint, a tibio-femoral joint with tears in one meniscus, and a tibio-femoral joint after meniscectomy. According to Pena et al., the minimal principal stresses corresponding to a compressive load at 0° flexion were obtained for the posterior zone of the medial meniscus and the corresponding region of the articular cartilage. The maximal contact stress in the articular cartilage after meniscectomy under an axial femoral compressive load was about twice that of a healthy joint [27]. This fact could partially explain the cartilage damage and degeneration that have been occurring after meniscectomy.
New Treatment Options
Meniscal Scaffold
To create the custom-made scaffold with definite pore dimension and consequently biomechanical features and the high biocompatibility of the whole tissues are important. Also, there are too many features important such as cell attachment. A biomaterial used as scaffold for meniscus tissue engineering purposes should present many features. The ideal meniscal scaffold should be (1) “cell-instructive”, promoting cell differentiation and proliferation if cell-seeded, or cell migration if cell-free; (2) “biomimetic”, mimicking architecture, tribology and mechanical features of the native meniscus; (3) resilient and resistant to withstand mechanical forces acting in the joint while cells produce ECM (extracellular matrix); (4) biocompatible, not evoking any foreign-body reaction also with its degradation products; (5) slowly biodegradable, allowing to be gradually replaced by biologic tissue; (6) open, with high porosity, allowing diffusion of nutrients and catabolic substances; and (7) easy to handle, to be sutured and to be implanted by the surgeon [35, 36].
With the ultimate goal of designing the ideal scaffold for meniscus tissue engineering, many biomaterials have been evaluated, both natural and synthetic [37]. Natural materials used to date are as follows: periosteal tissue [38]; perichondral tissue [39]; small intestine submucosa (SIS) [40]; acellular porcine meniscal tissue [41]; and bacterial cellulose [42]. While these tissues have high biocompatibility, they do not allow varying structure geometry and initial mechanical properties because some of them cannot be employed for tissue engineering techniques [37]. A more attractive strategy is represented by isolated tissue components like collagens and proteoglycans [43, 44]. They maintain the high biocompatibility of the whole tissues while allowing to create custom-made scaffold with definite pore dimension and geometry and, consequently, biomechanical features.
However, these scaffolds have usually low biomechanical properties and are characterized by rapid biodegradation, consequently not long enough to be completely replaced by the newly formed tissue [37].
On the other hand, polymer materials can be manufactured in custom-made shapes of any geometrical structure, porosity and biomechanical properties, according to the characteristics of the host tissue and the seeded cells. In particular, it has been shown that for optimal ingrowth and incorporation of a meniscal scaffold, macropore sizes must be in the range of 150–500 μm [45]. The biodegradation rate can be also modulated by acting on polymer composition. The most used synthetic polymers are as follows: polyglycolic acid (PGA) [46]; poly(L)lactic acid (PLLA) [47]; poly-(lactic-co-glycolic acid) (PLGA) [48]; polyurethane [49, 50]; polyester carbon [51]; polytetrafluoroethylene [52]; and polycaprolactone (PCL) [53]. Possible disadvantages of the use of synthetic polymers for tissue engineering purposes are the low cell-adhesive properties, since they lack the cell-adhesion domains normally present on natural macromolecules, and already mild foreign-body reaction occurring after implantation [54, 55]. In order to improve biocompatibility and biodegradability of polymer scaffolds, a biopolymer, such as silk fibrous protein, has been suggested [56, 57].
An alternative strategy is represented by the use of hydrogel materials. Their semi-liquid nature allows engineering anatomic geometries derived from medical imaging techniques, such as computed tomography or magnetic resonance, by the use of custom-printed moulds [58]. Auspicious results were reported with alginate [58, 59] and polyvinyl alcohol (PVA) [60, 61].
Briefly, both natural materials and synthetic polymers present advantages and disadvantages. Most importantly, no biomaterial demonstrated to be superior to the others in terms of supporting cell proliferation and tissue growth. No clear advantage has been shown in terms of biomechanical properties suitable for implantation in the knee joint. A possible solution is represented by combining them, in order to couple the high cell affinity and biocompatibility of natural polymers with the superior mechanical strength and practicality planted of synthetic polymers. This strategy has been recently evaluated in two large animal studies on partial and total meniscus tissue engineering with a hybrid material composed of PCL and hyaluronic acid (HA) with good results [62, 63].
Meniscus Prosthesis
Biomaterial prostheses could have great potential. They are easy to produce and are available in unlimited numbers. Material composition, physical structure and size can be controlled, and immunologic problems can be prevented. Prostheses have been made of reconstituted collagen, polyester–carbon fiber, fiber–teflon, and Dacron with polyurethane coating.
Klompmaker et al. showed that a meniscal replica can develop after implantation of a porous polymer prosthesis. When compared to meniscectomy, cartilage degeneration decreased [64].
Esposito et al. evaluated the ability of fibrochondrocytes preseeded on PLDLA/PCL-T [poly(L-co-D,L-lactic acid)/poly(caprolactone-triol)] scaffolds to stimulate regeneration of the whole meniscus. The aim of this study was to assess the usefulness of primary cultures of rabbit meniscus fibrochondrocytes grown on PLDLA/PCL-T (90/10) scaffolds for meniscus regeneration. At the end of the study, it has been shown that the PLDLA/PCL-T 90/10 scaffold has potential for orthopedic applications since this material allows the formation of fibrocartilaginous tissue, a structure of crucial importance for repairing injuries to joints, including replacement of the meniscus and the protection of articular cartilage from degeneration [65].
Studies have been reviewed of two types of scaffolds in the literature: CMI (Collagen Meniscus Implant—Ivy Sports Medicine GmbH, Germany); Actifit (Orteq, United KingdomGood). Clinical results have been documented both at histological and MRI evaluation. Safety and positive results have been shown for both scaffolds [66].
A.
The Menaflex CMI is a porous collagen-glycosamino-glycan (GAG) matrix of defined geometry, density, thermal stability, and mechanical strength [67, 68]. The CMI is composed of about 97 % purified type I collagen (the most commonly found protein in the body). The remaining portion of the CMI consists of GAGs (glycoseaminoglycanes), which include chondroitin sulfate and hyaluronic acid [67, 69, 70]. The type I collagen is isolated and purified from bovine Achilles tendons from animals originating in the United States. It is implanted in the joint and fixed with sutures to the host meniscus [67].
B.
The Actifit meniscal implant (Orteq Sports Medicine, London, UK) is a biodegradable, synthetic, acellular scaffold composed of aliphatic polyurethane. It has been designed to treat symptomatic, segmental defects of both medial and lateral menisci, thereby regenerate its biomechanical function and satisfactory clinical outcomes. It is a highly porous structure (approximately 80 %), allowing biological tissue ingrowth. In animal studies, removal of the material has been shown safely, as well as tissue ingrowth and vascularization [71].
A goal in the surgical management of meniscal tears or defects is to recreate a mechanically functional tissue in an attempt to halt disease progression. Use of scaffolds to facilitate tissue ingrowth into meniscal defects is one such option that is under investigation. Although not yet used clinically, cell-seeded scaffolds designed to be cultured in a laboratory setting prior to implantation have been developed for the treatment of meniscal defects [63, 72, 73.
< div class='tao-gold-member'>
Only gold members can continue reading. Log In or Register a > to continue
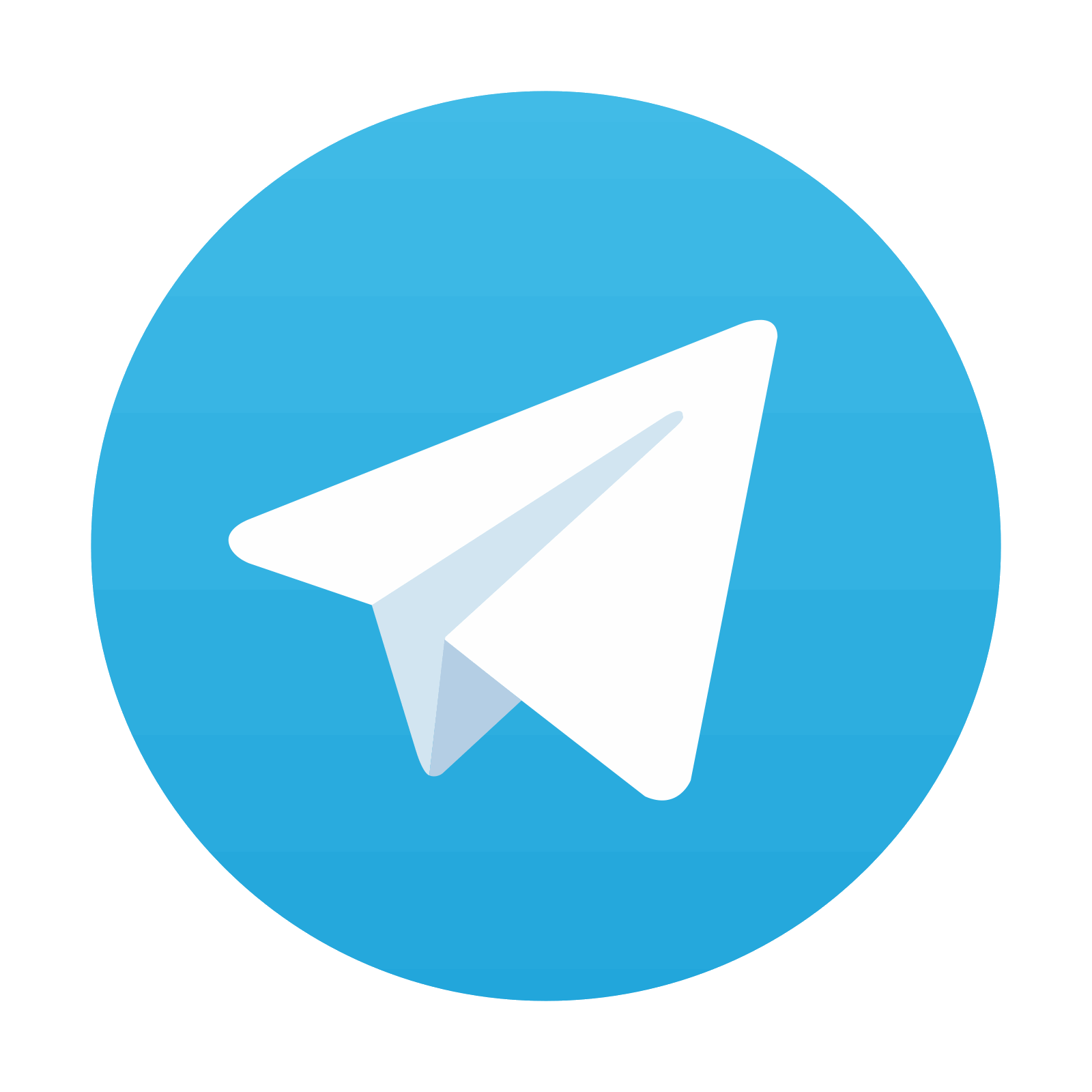
Stay updated, free articles. Join our Telegram channel
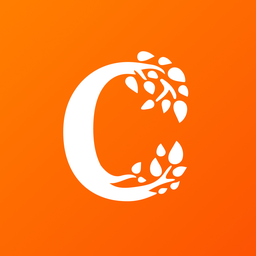
Full access? Get Clinical Tree
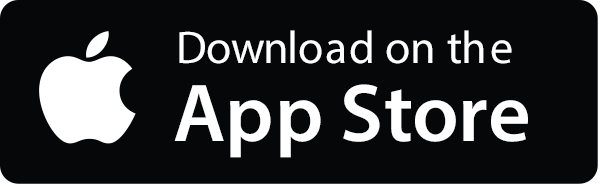
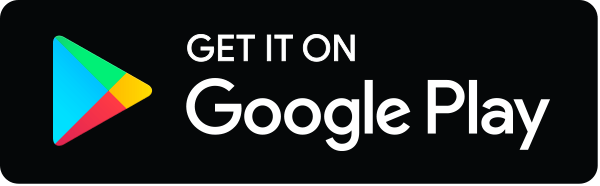