Fig. 15.1
Stem cells according to sources
Stem Cells According to Sources and Potentials
Embryonic Stem Cells (ESCs)
Embryonic stem cells (ESCs): Each cell of the blastocysts (inner cell mass) formed on day 5 after formation of the zygote is called an ESC. These cells are pluripotent and differentiate into all cells of the body. In addition, they have the advantage of the ability to expand and differentiate in vitro and can also be genetically modified. However, the clinical use of ESCs is hindered on a large scale because of high risk of developing cancer, and there are ethical and legal problems associated with the use of human embryonic stem cells [3].
In vitro studies with embryonic stem cells have been especially concerned with spinal cord injuries. Embryoid bodies were developed from ESCs and neuronal progenitor cells were then obtained with a protocol developed by David Gottlieb, PhD, of Washington University (St. Louis, MO, USA) [4]. Retinoic acid treatments resulted in nestin-positive neural progenitor cells that could be differentiated into neurons, astrocytes, and oligodendrocyte cells [5]. Obtained neural progenitors were marked and injected directly into the injured spinal cords of rats. Two weeks after transplantation oligodendrocyte, astrocyte, and neuron differentiations were observed. In addition, a better Basso, Beattie, Bresnahan (BBB) locomotor rating scale scoring was obtained in transplanted animals.
Despite the positive results of animal experiments with ESCs, the risk of forming tumors, immunological responses, use of short-lived experimental animals, and unavailability of human ESCs make clinical use of these cells impossible today.
Fetal Stem Cells (FSCs)
Fetal stem cells (FSCs) are obtained aborted fetuses, aged 5 to 9 weeks. These cells are pluripotent/multipotent and have self-renewal features. Because of these properties, they are an alternative to ESCs. Clinical use is limited, however, because of the risk of tumor formation. Appropriate sources of fetal tissue include donations following spontaneous abortion, but there are ethical issues that must be considered [6] (Fig. 15.2).
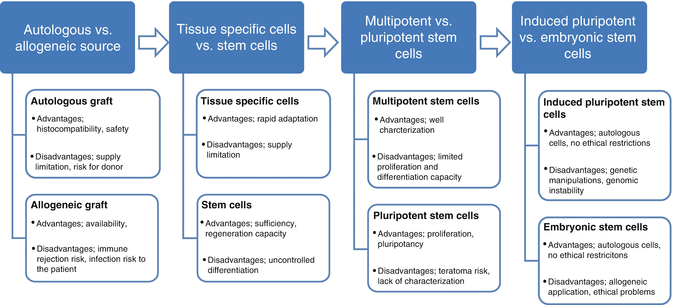
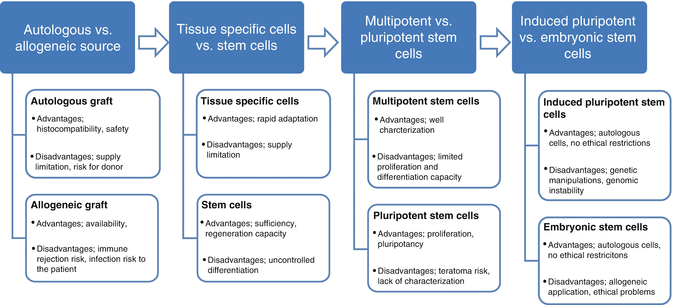
Fig. 15.2
Cell sources for tissue engineering: advantages and disadvantages
Adult Stem Cells (ASCs)
MSCs
General Characteristics of MSCs
General Characteristics of MSCs: MSCs, especially connective tissue-derived cells (bone, cartilage, fat, tendon, stroma), differentiate into many tissue cells (heart, liver, pancreas, nervous system cells) and/or synthesize soluble factors that contribute to tissue/organ regeneration. MSCs expand in vitro by protecting their stem cell properties and support hematopoiesis; when they are transplanted they are not rejected by immunosuppressive properties. Thus, these cells have possible applications in many clinical areas [7].
MSCs are the main cells of connective tissue. These cells were first identified in 1976 by Friedenstein [8], who, using fetal calf serum, showed that cell colonies are adhesive and morphologically resemble fibroblasts in bone marrow culture. These cells can differentiate into bone, fat, and cartilage cells. Previously, these cells were called colony-forming unit fibroblasts (CFU-F) and bone marrow stromal fibroblasts, but they are now known as mesenchymal stem/stromal cells.
Bone marrow, one of the richest sources of stem cells in organisms, hosts hematopoietic, endothelial, and mesenchymal stem/progenitor cells originating from the mesoderm [9]. Different studies indicate that an average of 2 to 100 MSCs have been identified in the 1 × 106 mononuclear cells (MNCs) in bone marrow aspiration. MSCs can be isolated from many tissues other than bone marrow. Enzymatic methods are used for the isolation of MSCs in solid tissues. MSCs can be isolated and expanded because of the adhesion properties from bone/periosteum, muscle tissue, dental pulp and maxillofacial tissues, liver, lipoaspiration materials, umbilical cord blood, cord stroma, placenta, amniotic fluid, and synovial fluid, and even stimulation through the peripheral blood. They have many properties such as adherence to plastic tissue culture dishes, showing fibroblastoid morphology, differentiation, and surface markers, and these features are independent of the tissues they were obtained from. These properties are substantially similar, but the differentiation capacity and functional characteristics may show some changes according to origin of tissue type [10].
Molecular Surface Properties of MSCs
In cultures with a heterogeneous cell population, MSCs can be distinguished from other cells by their cell surface molecules, which are different from other hematopoietic stem cells (HSC) and/or tissue-specific antigens. In contrast, being involved in adhesion, cell-cell and cell-extracellular matrix are known to be expressed high. However, a specific antigen has not been described for MSCs [11].
Molecular cell surface properties of bone marrow-derived and cultured MSCs have been studied in detail by flow cytometry. According to this technique, the hematopoietic antigen (e.g., CD45, CD34, CD14, CD11b, HLA class II, CD79α, CD19) positivity rate must not exceed 2 % in the cell population. However, stroma-associated antigens (CD73, CD90, CD29, CD44) must be positive.
According to the International Society for Cellular Therapy (ISCT) criteria, for the antigens CD105 (endoglin), CD73 (ecto-5′-nucleotidase), and CD90 (Thy-1), more than 90 % must be positive in an MSC population [10].
Secretory and Immunomodulatory Properties of MSCs
MSCs, which are found in various tissues as support cells, synthesize a large number of bioactive molecules, cytokines, chemokines, enzymes, and extracellular matrix proteins in relation to these features. MSCs release growth factors required for hematopoietic cells in bone marrow such as macrophage colony-stimulating factor (M-CSF), Flt-ligand, and stem cell factor (SCF). Furthermore, not only interleukin-6 (IL-6), IL-7, IL-8, IL-11, IL-12, IL-14, and IL-15 cytokines but also MSCs synthesize chemokines, including stromal-derived factor-1alpha (SDF-1alpha)/CXCL12 and monocyte chemoattractant protein (MCP)-1 [12].
MSCs transplanted into the injured area of the spinal cord have been shown to reduce apoptosis in neuronal cells [13]. Other studies have shown that neurotrophins such as brain-derived neurotrophic factor (BDNF) and nerve growth factor-β (NGF-β) are released by the MSCs that increase the life of the neuronal cells [14–16]. In addition, in vitro cocultures obtained from 25 different bone marrow MSCs and neuronal stem cells obtained from the cerebral cortex of mice showed that increased survival and migration properties have been observed for neuronal cells [17].
One of the most advantageous in vivo applications of MSCs is that immunogenicity of these cells is low and has inhibitory properties on the immune system. Besides these immune-regulating properties, they exert anti-inflammatory effects. They also prevent alloreactive T-cell activation and further reactions, inhibit activated B lymphocytes, and stimulate regulatory T cells. Thus, an immunosuppressive effect was shown resulting from the inhibiting lymphocyte proliferation in animals and humans. A dose-dependent stimulating effect probability is shown on the immune system in some circumstances [18]. In addition, the immunomodulatory effects of MSCs, anti-apoptotic factors, and angiogenic and antifibrotic properties are also available. These features help explain their role in wound healing [19]. MSCs also prevent scar tissue formation with their antifibrotic property in spinal cord injury and inhibit the axonal regeneration [19].
Differentiation Potential of MSCs
The most interesting feature of MSCs, especially for applications in regenerative medicine, is the potential to differentiate into a variety of cell types in appropriate microenvironments, including connective tissue. Several researchers have stimulated in vitro osteogenic, adipogenic, chondrogenic, and myogenic differentiation with appropriate signals and demonstrated generated hematopoietic stroma. In the field of regenerative medicine, “stem cell plasticity” is the ability of a cell to differentiate into tissues other than that from which it originated [10]. Myoblast cell [20] and hepatic [21], cardiac [22], renal [23], and neural cell [24] differentiations have been shown in the studies with bone marrow-derived stem cells.
MSCs migrate to sites of inflammation in the body and differentiate to damaged cells and are helpful in healing wounds. The same property is realized for transplanted MSCs in the damaged area. Rat bone marrow stromal cells transplanted to spinal cord injury patients differentiated into neurons [25] and astrocytes [26], and functional improvement was documented based on BBB behavioral rating scoring [27].
Contribution of MSCs to Repair Damaged Tissue
Effective mechanisms of MSCs for improvement in damage, differentiation to mature functional cells, cell function recovery after damaged cell-MSC fusion, cell-cell and cell-extracellular matrix relationship with MSCs, release of the soluble factors (growth factors, cytokines, chemokines, etc.), paracrine factors, and the enzyme or immunomodulatory, anti-inflammatory, anti-apoptotic, and angiogenic effects can be considered [28].
Migration is required of MSCs from their niche into the damaged tissues. The signal for migration of cells has been shown to come from the damaged tissue microenvironments. Soluble factors like SDF-1/CXCL12, MCP-1, and complement C3 fraction released in the damaged area are known to have important roles in migration [29].
In spinal cord injury, a major problem is damage to cells forming the layer of myelin (oligodendrocytes and Schwann cells) and the transmission of pulses in the myelin sheath surrounding axons [30]. In studies, MSCs have been shown to prevent myelin loss and to promote myelination in spinal cord injury. Akiyama et al. [31] showed that bone marrow stromal cells differentiate into cells that express myelin and a myelin layer reformed around demyelinated axons [31]. In this study, the pulse transmission rate of the demyelinated axons was increased compared with the control group, as determined by in vitro electrophysiological methods. These results were supported in other studies carried out by Akiyama. Bone marrow-derived stromal cells differentiated especially into Schwann cells have been proven in another study [32].
Tracking of MSCs in Tissue
Clinical benefits were obtained with MSC infusion or tissue implantation in in vivo applications, but the fate of these cells after infusion, migration to damaged tissue and placement or accumulation in that area, proliferation, and differentiation features have not been studied in detail. In most studies, after cell infusion, difficulties are encountered in determining the presence of cells in tissue or blood.
Demonstration of different sex cells of the donor or use of species-specific markers (e.g., beta-2-microglobulin for human cells in animal tissues) are among the methods applied for the purpose of tracking MSCs in tissue. Tagging cells with fluorescent dye is one of the easiest methods of tissue tracking [33]. Temporary fluorescent markers such as CFSE, DiL, and DiO can also be used for short-term experimental studies [34]. Genetic marking with green fluorescent protein (GFP) is the preferred method because it is permanent. In vivo tracking of luciferase-labeled MSCs with a bioluminescence device and labeling with superparamagnetic iron oxide nanoparticles and imaging in vivo under magnetic resonance are other commonly used methods [35].
Advantages of Clinical Use of MSCs
The advantages of MSCs in clinical practice are as follows:
Because of differentiation of connective tissue stromal cells, MSCs provide support related to the development and function of tissue cells.
With their differentiation capacities, MSCs can become mesenchymal tissue cells such as muscle, fat, bone, cartilage, stromal cells, tendons, and ligaments and have differentiation capability for other tissue cells (transdifferentiation; neurons, hepatic, pancreatic, etc.) [36].
MSCs are capable of fusion with damaged cells.
MSCs contribute to the repair of damaged cells and tissues by growth factors, cytokines, and chemokines such as exosomes directly or via secreted soluble factors.
With migration characteristics, MSCs have access to damaged tissue.
MSCs are immunosuppressive and nonimmunogenic and because of these characteristics do not require HLA compatibility for clinical use.
Induced Pluripotent Stem Cells
Induced pluripotent stem cells (iPSCs) are cells that have gained pluripotency characteristics by the application of various methods and factors. The term iPSC was used for the first time in 2006 by Shinya Yamanaka of Kyoto University. Yamanaka et al. obtained ECS-like cells with retroviral transfection of four pluripotency genes (Oct4, Sox2, KLF-4, c-Myc) from skin fibroblast cells. Yamanaka and John Gurdon, who laid the foundation of cloning, showed terminally differentiated cells can gain pluripotency and were awarded the Nobel Prize for medicine in 2012 [38].
In the years since, the number of studies with iPSCs has increased rapidly. Improvements such as increased efficiency of forming cells, use of tissue-specific multipotent stem cells rather than terminally differentiated cells, and more convenient methods of clinical use instead of viral transfer increase the chances of clinical use of these cells. The greatest advantage of these cells is the absence of immunological incompatibility problems. Genetic corrections derived from pluripotent stem cells can be performed and can be differentiated to desired cells. Although iPSCs have many advantages compared with embryonic cells, there are challenges that must be overcome before clinical application. First, using nonviral methods, iPSC efficiency should be improved. In addition, the methods used must not create susceptibility to tumor formation. Xenogenic factors should not be used to avoid immunological reactions [39].
iPSCs are used in orthopedics to differentiate into bone and cartilage cells. iPSCs are expanded on tissue scaffolds and differentiated into osteogenic and chondrogenic cells. Prepared cell-containing tissue scaffolds are injected into immunosuppressed mice, and bone and cartilage tissue formation are monitored. In addition to direct differentiation, iPSCs can be differentiated into MSCs before bone and cartilage cell differentiation. Thus, it is argued that a more controlled protocol be followed [40].
Tissue Engineering
The main goal of tissue engineering is to create tissues or organs for use in case of loss or damage. Tissue engineering includes four stages: the first using only biomaterials; the second using only cells (directly or with genetic modifications (gene therapy)); the third using biomaterials with adhesion and expansion biosignals; and the fourth using biomaterials, biosignals, and cells together. For the purpose of cell growth for guiding new tissue or organs and providing mechanical support, three-dimensional scaffolds can be produced from biomaterials. In addition, bioreactors can be used to mimic a tissue microenvironment [41].
Tissue scaffolds are produced to mimic extracellular matrix and they should provide a suitable surface for appropriate cell growth and adhesion, mechanical strength, and effective environmental and tissue interactions. Selection of biocompatible and biodegradable materials is important [41].
Cartilage, bone, and tendon regeneration is often studied in tissue engineering applications. Scaffolds with different properties for each application (size, three-dimensional structure, surface pattern, porosity, mechanical strength, etc.) should be used.
A tissue scaffold should have the following properties:
It should promote cell adhesion and survival.
It must have suitable porosity to support the expansion of the cells and formation of extracellular matrix.
Its structure should permit nutrients to reach the cells and metabolites to be removed.
Tissue scaffold degradation rate and new extracellular matrix formation rate must be the same.
Cellular Therapy in Orthopedic Research
Articular Cartilage
Despite being prone to damage, the repair capacity of articular cartilage is low. Traumatic injury causes arthritic changes over the years. The treatment protocol includes infiltration or introduction of bone marrow stem cells to the damaged area with penetration of the subchondral bone so that localized cartilage is repaired. The microfracture technique is frequently used, however, it results in formation of a fibrous structure that has weak mechanical properties compared with normal cartilage. Nevertheless, use of this technique for the treatment of osteochondritis dissecans or traumatic osteochondral lesions has shown good outcomes at mid-term follow-up [43].
More recently in clinical applications, the regeneration of normal articular cartilage with autologous chondrocyte implantation (ACI) has been achieved [44]. Chondrocytes, mesenchymal stem cells, periosteum-containing chondroblasts, and osteochondral autograft (mosaicplasty) transplantation to articular cartilage defects of animal models provided better recovery compared with controls. Mosaicplasty showed better results compared with periosteal grafts [45].
Bone
Trauma and certain pathological conditions may cause large losses that require bone tissue or patch transfusions with the aim of preserving the structural integrity. The use of autologous or allogeneic bone graft can cause morbidity and infection. Mouse, rat, rabbit, dog, and human bone marrow cell isolation and expansion methods have improved, and bone formation was observed in ectopic implantation with ectopic hydroxyapatite or suitable carriers [46]. There is also expansion of bone marrow osteoprogenitor cells. Therefore, the use of autologous human stromal progenitors has been developed for the regeneration of large bone defects [47].
< div class='tao-gold-member'>
Only gold members can continue reading. Log In or Register a > to continue
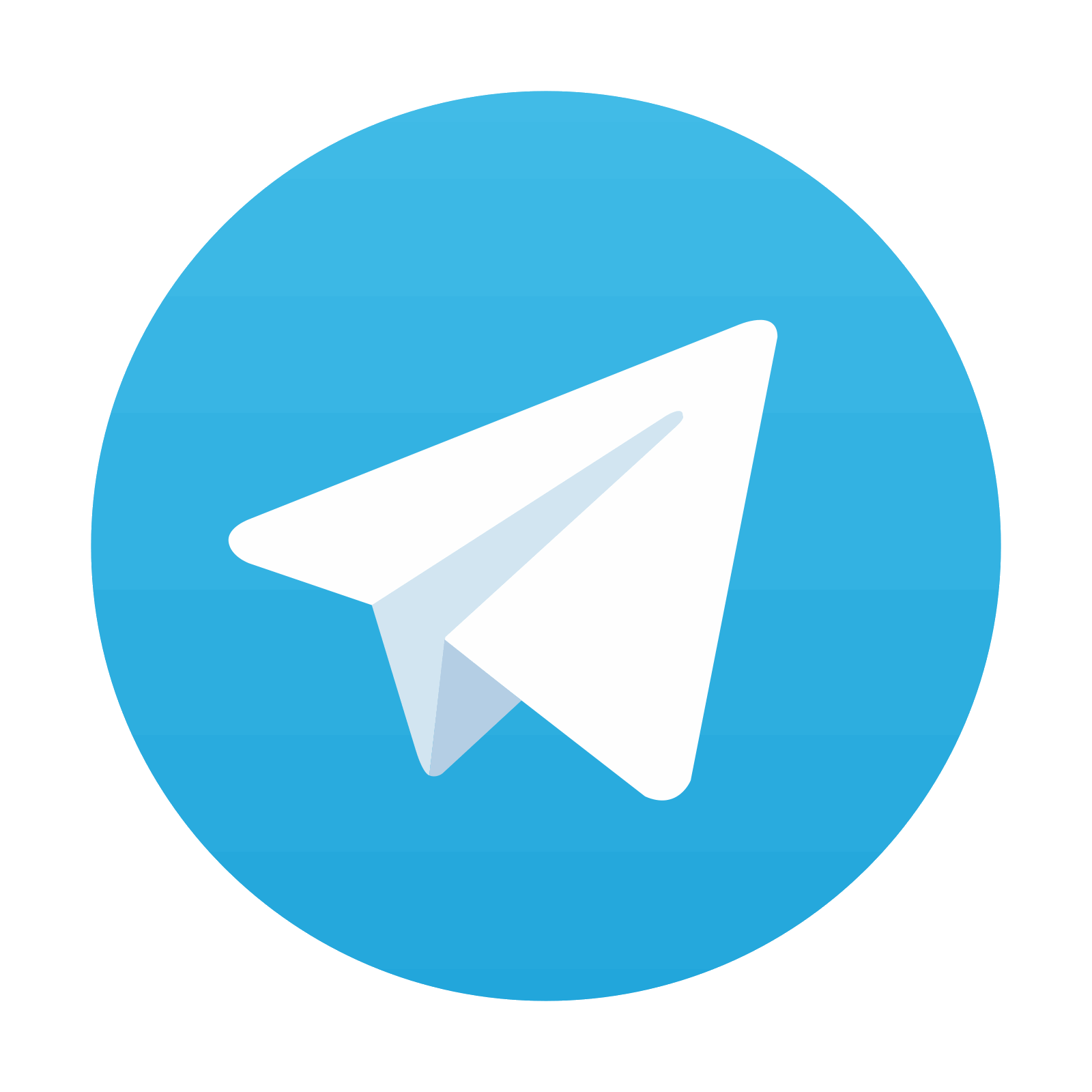
Stay updated, free articles. Join our Telegram channel
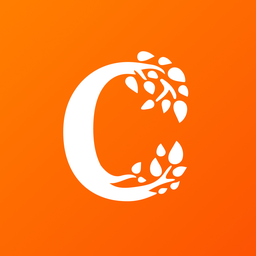
Full access? Get Clinical Tree
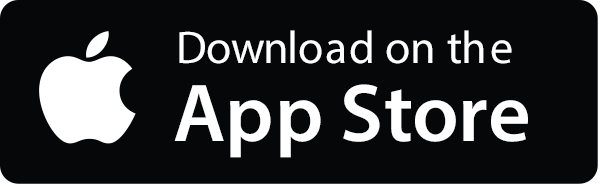
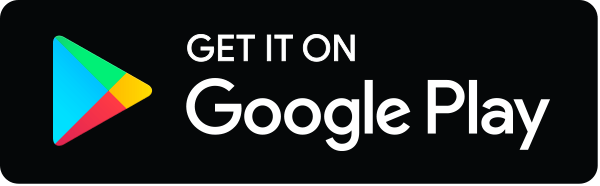