Tribology of the Artificial Hip Joint
Markus A. Wimmer and Michel P. Laurent
Key Points
• Hip wear is a major factor in the service life of hip prostheses.
• Significant progress is still required to reduce wear and its effects on the host tissue.
Introduction
Tribology is the science and technology of interacting surfaces in relative motion. It includes the study and application of the principles of friction, wear, and lubrication. Friction is a natural phenomenon in our daily lives and causes wear of bodies in contact. Although Leonardo da Vinci (1452–1519) and Guillaume Amontons (1663–1705) already recognized and formulated the basic principles of friction, the underlying mechanisms of many effects of friction and wear are poorly understood. Lubrication of contacting bodies helps to reduce and control detrimental consequences of friction and wear. In this chapter, we will present the current understanding of tribology in the context of prosthetic wear, with a focus on hip implants. However, the principles also apply to any other articulating joint replacement.
In the artificial hip, wear and the consequences of wear continue to be an important cause of implant failure. Billions of wear particles generated annually can migrate to the periprosthetic tissue and cause localized chronic inflammation and resorption of bone adjacent to the implant.1,2 This mechanism, called osteolysis, may lead to subsequent implant loosening and failure and is discussed in detail in Chapters 11 and 12.
Because of the biological complications of wear debris generated by prosthetic joints, it is important to identify precise and purposeful measures for wear reduction. Knowledge of the multifactorial nature of wear and accurate modeling of in vivo conditions within the laboratory will allow us to overcome the simple trial-and-error methods of the past and will help reduce risks for the patient.
Therefore, it is essential to analyze surfaces that have been worn under the actual “operating conditions” to learn about the influencing factors of the system and replicate them satisfactorily in a bench test.
This chapter covers the major aspects of tribology related to the articulating surfaces of hip prostheses. First, the definitions of terms associated with a tribological system are given, with particular emphasis on the various wear mechanisms, wear modes, and lubrication regimes. The hip bearing is then presented as a tribological system, with specific wear modes and mechanisms, outputs (material loss, heat, and sometimes sound), and lubrication regimes. The wear characteristics of hip bearing couples as a function of geometry and bearing materials are discussed. This is followed by a section on wear testing procedures, covering screening tests for materials, and full-fledged tests of hip bearings in simulators. The chapter ends with a list of current concerns and future directions associated with hip wear, which include uncertainties in the long-term wear behavior of highly cross-linked polyethylenes, improving the wear of metal-on-metal bearings, developing new materials and coatings for bearing surfaces, improving wear testing of prosthetic hip joints, and developing virtual wear testing that would complement actual wear testing.
Basic Science
Definition of Terms
A tribosystem consists of four principal elements: a body, a counterbody, an interfacial medium, and an environment (Fig. 3-1). The relative kinematics of the bodies, the contact load and loading profile, and the ambient temperature define the input variables of the system. The mechanical function of motion with load bearing that characterizes every tribosystem is always accompanied by some loss of energy, mostly in the form of heat (≈90% of the introduced energy), sound, and wear. Loss of material generated by the wear component is influenced by many factors, including general bulk properties of the articulating bodies, their surface characteristics (e.g., roughness, hardness, surface energy), and system conditions, such as the lubricant, the relative motion of the bodies, and the loads transmitted.
Figure 3-1 General description of a tribosystem, which consists of four elements: the two bodies in contact, the interfacial material, and the environment. All these elements can affect each other and change the mechanism of interaction.
Knowledge of the contact conditions between interacting bodies is important for the understanding of wear mechanisms. On the microscopic level, all surfaces have an intrinsic roughness, even those that appear perfectly smooth (see Fig. 3-1). Hence, contact between articulating surfaces is established only on asperities, yielding many tiny contact spots, so that the real contact area is significantly smaller than the apparent contact area (Fig. 3-2). This distinction between real and apparent contact areas is a key concept in tribology. During motion, these tiny contact locations are deformed elastically and/or plastically within a chemically challenging environment. Particles are then created by mechanically or chemically dominated wear mechanisms.
Figure 3-2 Apparent and real areas of contact established on asperities.
Currently, four major wear mechanisms are known and distinguished: (1) abrasion; (2) surface fatigue, which involves microscopic crack initiation and propagation; (3) adhesion; and (4) tribochemical reactions, which involve primarily chemical processes. These four mechanisms are described in greater detail in Box 3-1. Depending on the chemical reactivity of the bearing material, chemical bonding between articulating materials (adhesion) or with surrounding agents (tribochemical reaction) can occur. In simulating the wear of hip prostheses within the laboratory, it is essential to replicate the in vivo wear mechanisms, not just the observed wear rates (the wear loss per cycle or unit time). Key indicators of the validity of laboratory simulations include the surface appearance and morphology of the worn surfaces, which must resemble those of explanted components, and the shapes, size distributions, and chemistry of wear particles generated during laboratory testing, which must duplicate those collected from periprosthetic fluid and tissue.
The wear mode is the particular dynamic configuration of body, counterbody, lubricant, and environment that generates wear in the tribosystem. Eight common wear modes are depicted and defined in Figure 3-3, including sliding wear, rolling wear, and three-body abrasive wear. In the hip joint, for example, wear between the head and the cup occurs as the result of sliding wear. In the presence of bone cement particles, the wear mode shifts to three-body abrasive wear. These two-wear modes trigger profoundly different wear mechanisms, and thus generate different types of wear, which will be explained in detail later. As a consequence, a difference in volume loss is generated. Knowledge of the wear mode is important for proper replication of the wear situation in the laboratory (e.g., in the case of the hip joint, the daily activity profile of a patient must be emulated). It should be noted that a wear mode is not a steady-state condition, and that a shift between modes may occur. For example, worn carbides generated from metal-on-metal sliding wear may change the wear mode to three-body abrasion, as the particles released from the bearing surfaces actively participate in the tribological process.
Figure 3-3 Schematic diagram of different wear modes and possible wear mechanisms in a tribological system. Tribological operating conditions are shown pictographically, followed by a description of the wear mode. Possible wear mechanisms are listed in the outer boxes for each mode, with the prevalent ones shown in bold.
Each wear mechanism generates a characteristic wear appearance, also known as a “wear pattern” or “wear damage,” observed through visible changes in surface structure (texture and shape) that occur as a consequence of wear. Examples are shown in Figure 3-4.
Figure 3-4 Typical appearances of the four major wear mechanisms (from left to right). A, Abrasion: scratches and grooves on a polyethylene cup. B, Adhesion: transferred polyethylene flakes. C, Surface fatigue: intergranular fracture in the high wear region of a ceramic cup. D, Tribochemical reactions: organo-metallic deposit on the head of a metal/metal articulation.
Friction is the introduction, transformation, and dissipation of energy. Surface asperities become elastically or plastically deformed when they come in contact (or interlock) with asperities of the countersurface (see Fig. 3-1). Another contribution comes from the adhesion of surface atoms and molecules of the body and counterbody.
Lubrication can reduce wear and friction. Both deformation and adhesion contributions to friction can be significantly reduced by lubrication. The extent of fluid film formation plays an important role in the wear process of artificial joints and has been described for technical bearings using a partial differential equation known as the Reynolds equation, derived from the general Navier-Stokes equations for laminar fluid flow.3 Richard Stribeck (1861-1950) developed the groundwork for a quantity known as the lambda ratio (λ), which is the thickness of the lubricating fluid film relative to the surface roughness of the contacting materials.4 The higher the value of lambda, the greater the thickness of the fluid film relative to the height of the asperities. The value of λ increases with the viscosity of the lubricant and the sliding velocity, and decreases with the load on the interface and the roughness of the mating surfaces. The value of λ also depends on the local gap geometry, because formation of a fluid film during sliding motion requires that the mating surfaces form a convergent gap (i.e., a slight wedge). During sliding, the fluid is entrained into the wider end of the wedge and gets partially trapped, forming a pressurized film that supports load. In a hip bearing, the slight difference in the head and cup radii will naturally produce such a convergent gap. The λ ratio is used to estimate the occurrence of three distinct lubrication regimes, as shown in Figure 3-5. These include (1) λ < 1, a region of boundary lubrication in which the asperities of the two articulating surfaces are in contact and the lubricant reduces resistance to relative motion between counterfaces by chemical and physical processes; (2) 1 < λ < 3, a region of mixed lubrication where parts of the surfaces are separated by the lubricant but isolated surface points are still in contact. In this context, the importance of well-polished surfaces is apparent: the higher a single large asperity protrudes through the lubricating film, the longer it will remain in contact with the other surface; and (3) λ > 3, a region of hydrodynamic lubrication where a full fluid film covers the asperities, completely separating the articulating surfaces. Although the friction coefficient is relatively low in this region, it increases with film thickness because the ability of the film to support load (FN) decreases faster than the corresponding reduction in viscous drag (FT), so the friction coefficient, which equals FT/FN, increases.
Figure 3-5 Coefficient of friction in sliding contact as a function of the specific lubricant film thickness.
The Hip Bearing as a Tribological System
In this section, we will apply the terms explained previously to the artificial hip joint. Because of the complex nature of tribology, wear at the hip cannot be reduced to a material property but is determined by the characteristics of the system. Such a system (compare with Fig. 3-1) consists of the acetabular liner and the femoral head, which are the contacting bodies, the fluid that interacts between the two bodies, and the surrounding soft tissue, with the latter defining environmental conditions such as ambient temperature and gas concentrations. These characteristics of the system, as well as the loads and motions that occur during daily activities, should be known for proper understanding and modeling of wear processes.
Wear Mode and Mechanisms
The wear mode in the human hip joint can be designated as multidirectional sliding wear—multidirectional because the wear tracks form quasi-elliptical paths, which cross each other during the cyclical motion of gait.5 Crossing of wear tracks accelerates the formation of particulate debris6 and thus is an important concept of hip wear. In addition, McKellop7 has defined four modes of hip wear based on the conditions under which the joint functions in vivo: (1) regular (sliding) wear; (2) impingement (impact) wear; (3) three-body abrasive wear; and (4) backside (fretting) wear. They are briefly described under “Testing Procedures” (Box 3-3, Item 5). During regular sliding wear, all known major wear mechanisms—adhesion, abrasion, surface fatigue, and tribochemical reactions—may act at the same time (see Fig. 3-3). Therefore, to tribologically improve an artificial bearing, it is important to identify the mechanisms that dominate the wear behavior. This has been done through retrieval analysis and identification of characteristic wear appearances. Based on the observed wear types, all four major wear mechanisms are found to be acting, and this has been documented in an extensive body of literature. Examples are presented in Figure 3-4.
System Output
Material loss is the most relevant system output (see Fig. 3-1) for orthopedic applications. The characteristics of wear particles are particularly important in the context of wear-induced osteolysis. It has been shown that the tissue reaction depends on the size, shape, and composition of the particles generated.8,9 A comparison of particle images (Fig. 3-6) reveals that different types of polyethylene result in different particle size distributions.10 For instance, in some cases, significantly more particles are released even though the overall quantity of wear debris may be smaller. Conversion of current wear rates and particle sizes predicts that 0.2 − 2.0 × 1012 particles are generated per milligram of debris, corresponding to the generation of approximately one hundred million (!) wear particles per step.11
Figure 3-6 Typical polyethylene wear particles from (A) conventional and (B) cross-linked ultra-high-molecular-weight polyethylene (UHMWPE).
Despite the relevance of wear, most of the dissipated energy of the system is transformed into heat. The clinically observed heat generation of hip endoprostheses12 is a direct result of the described microscopic process. In cases of couples with very small contact areas, as in metal-on-metal bearings, local temperatures can reach between 60° C and 80° C for a few milliseconds.13 These temperature changes can initiate chemical reactions that generate reaction products and films on the acetabular and femoral bearing surfaces.14 This phenomenon explains the higher starting torque measured for these metal-on-metal pairings,15 which stresses the prosthesis-bone interface. In large diameter prostheses, this start-up torque can reach clinically relevant values and in unfortunate circumstances can contribute to loosening of the artificial device.16 From a wear perspective, tribochemical reactions are positive. Transformation of the original surface into a hybrid material consisting of nanometer-sized metal crystals, oxidized wear debris, and organic matter from the interfacial synovial fluid17 may be similar to the action of antiwear additives in high-performance lubricants used in race car engines. Here, additives form surface films that protect the underlying material, making them more durable and reducing their wear rates.18
Lately, audible sound as a system output has come under scrutiny for hip arthroplasty applications. Cases of “squeaking” of ceramics/ceramics pairings19–21 have made their appearance in the orthopedic literature. The squeaky noise has been related to stick slip phenomena,22 which occur as the result of roughening of bearing surfaces. The exact cause of hip squeaking, however, remains unclear and is presumably a multifactorial phenomenon that could involve component neck-cup impingement, microseparation, and subluxation. Debate over whether squeaking in ceramic hips is a cause for concern is ongoing.
Lubrication Regime
Ideally, as reviewed in Figure 3-5, the lubricating film completely separates the two articulating elements. This scenario requires a large contact area, sufficiently high relative velocities, and sufficiently smooth surfaces. One application of these effects is the so-called large diameter prosthesis. Theoretically, the combination of a large femoral head (leading to high relative velocity), a small clearance between ball and socket (yielding a large lubricated area), and smooth bearing surfaces will facilitate hydrodynamic lubrication and thus yield a long-life implant without wear. However, theoretical calculations by Dowson23 showed that these effects are limited to the geometries of very few of the prostheses that are currently available. Nevertheless, clinical studies by Daniel and associates did not report significant differences in whole blood and urinary levels of cobalt and chromium between a theoretically low-friction group and a control group.24 It is possible that the daily activity profile of patients, low walking speeds, and many start/stop activities could have masked differences between groups in terms of wear under steady-state conditions.15
In addition, a small but significant deformation of the relatively thin metal socket may occur during implantation and physiologic loading of the pelvis (e.g., pinching effects around the equator). These deformations are considered in theoretical calculations25,26 but are not simulated in wear tests. These observations could explain a study conducted by DeHaan and colleagues27 on a marathon runner who had received a large diameter prosthesis: during the competition phase, a distinct increase in chromium urine concentration was found, which can occur only when the articulating components are moving in the mixed lubrication regime. Higher pelvis deformation and loads during running compared with those during walking may be responsible for this observation. Consequently, it must be assumed that all currently available implants interact under boundary or mixed lubrication conditions during physiologic loading to generate wear particles. The biological reactions that these particles elicit within the host will be reviewed in Chapters 11 and 12.
In this context, the question arises as to why in a natural hip joint, which is exposed to similar biomechanical boundary conditions, such wear processes do not occur. In the past, this difference was attributed primarily to elastohydrodynamic (EHD) processes. It was assumed that, in contrast to hard implant materials, the relatively soft cartilage is smoothed by the pressure of the lubricating film, reducing the effective height of protruding peaks. Under these conditions, it was assumed that a thinner lubricant film would be sufficient to separate the articulating layers without contact between the underlying surfaces. However, recent studies by Caligaris and coworkers28 have shown that the interstitial fluid pressure of cartilage is sufficient to reduce friction and wear substantially, which is especially important during starting processes in which EHD does not apply. Glycosaminoglycan is particularly important for this phenomenon. This molecule prevents rapid diffusion of fluid from the cartilage matrix, forcing 90% of the joint contact load to be carried by water molecules. In addition, special proteins, such as lubricin, within the synovium and the lamina splendens of the articular cartilage contribute to the absence of cartilage wear even in regions of mixed friction. Despite significant progress made in the understanding of articular cartilage performance, the development of comparable artificial materials remains challenging.
Wear in Hip Bearings
Because of the multifactorial nature of tribology, the relevance of results obtained in wear experiments using wear simulators should always be interpreted with great care. Nevertheless, the importance of tribological experiments for the development of new materials and revolutionary implant geometries is without question. For instance, Harry Craven29 was able to convince his boss, Sir John Charnley, who had considered the experiments a waste of time, of the suitability of ultra-high-molecular-weight polyethylene (UHMWPE) as a gliding component material and to replace the material Teflon.30 Craven used a pin-on-disk testing device manufactured from scrap, a device that would now be considered outdated. Nevertheless, use of this device facilitated the development of a milestone in endoprosthetics.
Approximately 6 years later (1966), Duff-Barclay started using the first hip simulator. Via several developmental stages, including Stanmore simulators (MKI and MKII), Munich simulators (Ungethüm 1 and 231), and Leeds simulators,32 new hip simulators were developed that meet current testing norms of the ISO (Standards 12424-1 and 14242-3). Although these tests exclusively simulate a normalized walking gait cycle (1 to 1.5 million movement cycles are assumed to reflect 1 year in vivo33), they have nonetheless provided major insights into wear processes in hip implants. Other activities of daily living, such as stair climbing or rising from a chair, are not considered, nor are events entailing high loads such as subluxation, start-stop movements, and neck-cup impingement.
In general, polyethylene wear increases by approximately 3% to 10% with each additional 1 mm in ball diameter because of increasing sliding distance and frictional area.34,35 Consequently, the risk of revision for a 33-mm ball size has been regarded as threefold compared with that for a 22-mm ball size for conventional polyethylene.36 Taking into consideration all ceramic-polyethylene pairings with a 28-mm diameter tested by a certified testing laboratory (Endolab GmbH, Rosenheim, Germany) into 12 groups of 3 pairings each during the past decade resulted in a median wear rate of 19.4 mg per million movement cycles (Fig. 3-7). The standard deviation was 6.8 mg per one million cycles, with values ranging from 7.8 to 29.8 mg per million cycles. Still, the worst 28-mm diameter pairing had a lower wear rate than the best 55-mm diameter pairing.
Figure 3-7 Steady-state wear rates of the most commonly used material combinations in hip bearing couples. Each dot represents the average of three bearings of the same design and manufacturer on a hip simulator according to the International Organization for Standardization (ISO) 14242-1. (Data from repository of Endolab.)
Wear rates of cross-linked polyethylene are in the range of 10% to 50% of those of conventional polyethylene.37,38 These low wear rates have been confirmed in many clinical studies.39–41 Likewise, studies by Endolab have shown wear in the range of a few milligrams per million loading cycles (see Fig. 3-7). In simulation experiments, all types of polyethylene absorb relatively large amounts of fluid from the surroundings, resulting in underestimation of weight loss through wear. In the past, these underestimations gave rise to the euphoric but scientifically unsound assumption that cross-linked polyethylenes are completely resistant to wear.37
Since the development of first-generation cross-linked polyethylenes, second-generation cross-linked polyethylenes have included additives such as vitamin E and altered manufacturing parameters. These additives primarily act as radical traps and allow the quenching of free radicals generated by irradiation without the need for thermal processing at temperatures that degrade the strength of the polymer. Removal of free radicals is beneficial in decreasing the rate of oxidation of polyethylene components and the risk of catastrophic embrittlement in vivo. Each group of polyethylenes with additives can be separated into subgroups42 with a range of possible material parameters. Because of these variations, large variability in wear rates is observed for cross-linked polyethylenes, and generalization of wear rates for these materials is not possible. In addition, aspects of the in vivo long-term stability of these materials cannot be generally addressed. However, because of the reduced fracture toughness of some formulations of cross-linked polyethylenes, enhanced protection against in vivo oxidation is especially important.
As was discussed previously, the “squeaking” of ceramic/ceramic pairings might be related to a subluxation problem with specific implant geometries. Subluxation was initially recognized in revised pairings43 and later included microseparation as an add-on for hip simulator tests.44 This helped to re-create areas of “stripe wear” in simulator testing that resembled those of ceramic/ceramic retrievals, namely, elongated zones with a dull appearance due to roughening of the surface. It is no surprise that such microseparation conditions produced considerably higher wear rates (typically one magnitude above those values shown in Fig. 3-7). Squeaking noises, however, could not be generated in the laboratory consistently. The reasons for this are currently under close investigation.22,45
Metal-on-metal bearings have regained popularity during the past decade. Until recently, 30% of all newly implanted hip joints in the United States were metal/metal articulations. Worldwide, its market share is approximately 10%. Although this material combination is attractive because of its low volumetric wear rate (see Fig. 3-7), it gained its popularity primarily because it provided new possibilities in surgical procedures using hip resurfacing rather than total hip replacement. Proper tribological behavior of metal-on-metal joints relies on the establishment of tribochemical reaction films.17 The creation of such films is sensitive to surface chemistry and texture, as well as contact pressure and lubricant constituents (i.e., organic molecules that adhere to surfaces). Hence, alloy microstructure, bearing dimensions, tolerances, and machining quality all may contribute to the large variability in wear rate seen with this combination (see Fig. 3-7). In vivo, surgical variation in implant positioning and the biomechanics of the patient are additional factors that need to be addressed. Also, variation in synovial fluid composition, as seen after osteoarthritic disease and/or menopause, may contribute to the wear outcome. This complex dependence on multiple factors may explain the variability in wear performance of metal-on-metal joints, including recent reports of higher than expected clinical wear rates and complications.46–48 Therefore, to make this bearing combination more reliable for clinical use, strategies should be developed to control and stabilize the formation of tribochemical reaction films. Because these films are the result of the combined action of corrosion and wear, their study is best performed within the scope of the newly established field of tribocorrosion.49,50
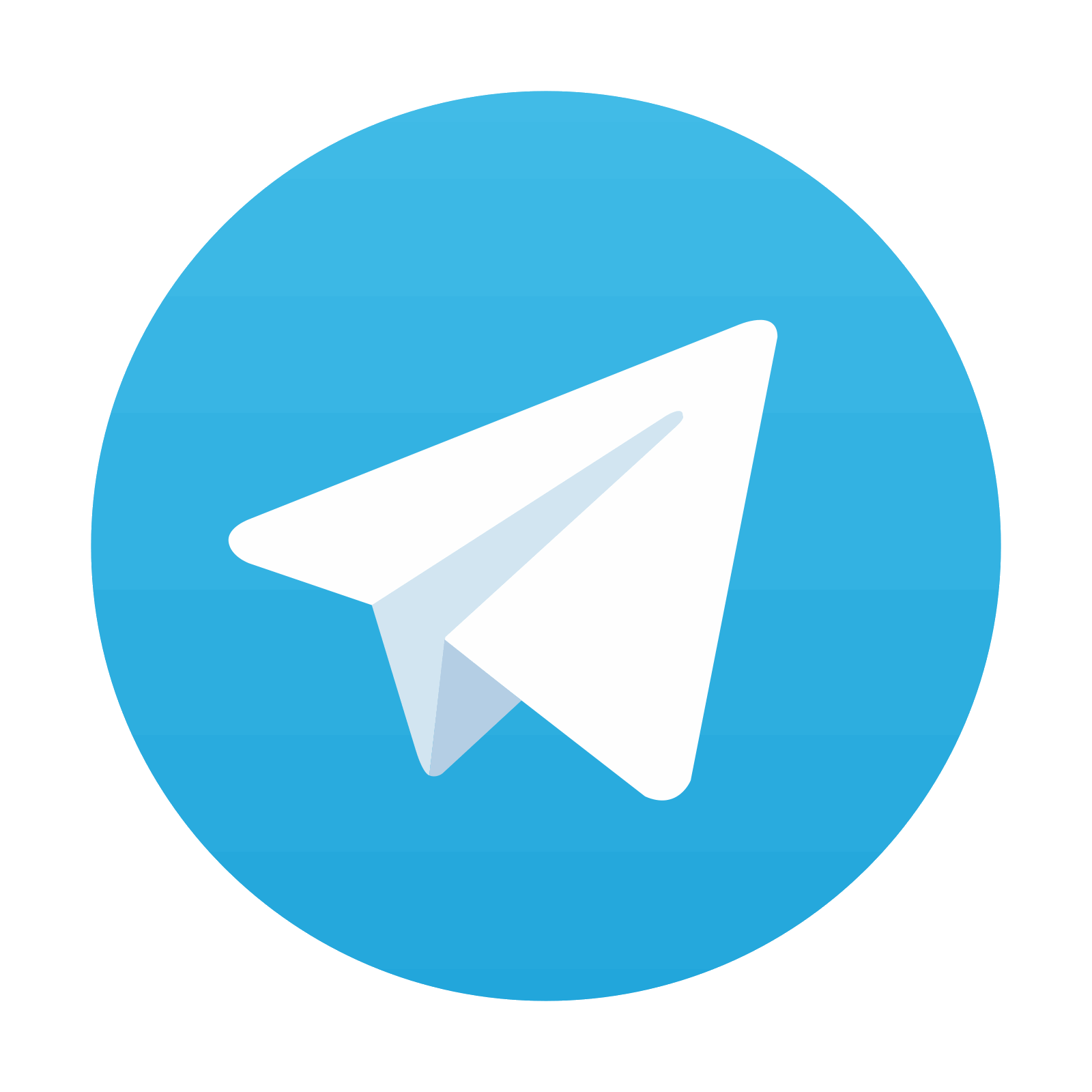
Stay updated, free articles. Join our Telegram channel
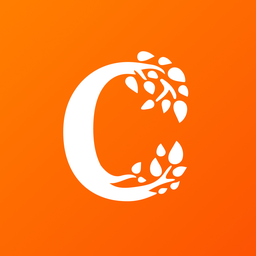
Full access? Get Clinical Tree
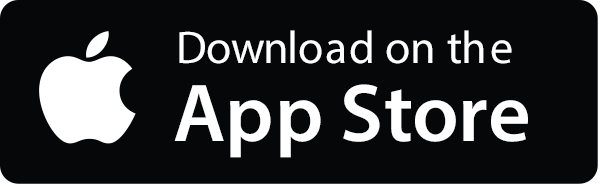
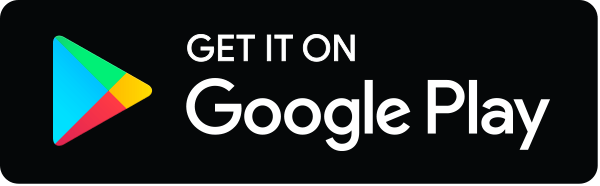