, Paul Santerre2, Eric Massicotte3, 4 and Mark Hurtig5
(1)
Department of Pathology and Laboratory Medicine, Mount Sinai Hospital, University of Toronto, Toronto, ON, Canada
(2)
Institute of Biomaterials and Biomedical Engineering, University of Toronto, Toronto, ON, Canada
(3)
Department of Neurosurgery, Toronto Western Hospital, Toronto, ON, Canada
(4)
Department of Surgery, University of Toronto, Toronto, ON, Canada
(5)
Department of Clinical Studies, Ontario Veterinary College, University of Guelph, Guelph, ON, Canada
26.1 Introduction
26.3.1 Annulus Fibrosus
26.3.2 Nucleus Pulposus
26.3.3 Cartilage Endplate
26.5.1 Cells
26.5.2 Scaffolds
26.5.3 Growth Factors
Abstract
In an autopsy study, 97 % of individuals 50 years or older had histological evidence of intervertebral disc degeneration, a disease process that involves the annulus fibrosus, nucleus pulposus, and cartilaginous endplate (Miller et al. 1988). The back pain that can develop as a result of this disease has a lifetime prevalence of up to 80 % (Manchikanti et al. 2009; Takatalo et al. 2011, 2012). Approximately 1 in 50 Canadians becomes disabled by back pain which is responsible for 40 % of all workplace absences (Iron et al. 2004; Lee 1994; Rapoport et al. 2004). Although rarely life threatening, the annual total costs in 2002 in the USA as a result of back pain were over $100 billion (Asche et al. 2007; Dagenais et al. 2008; Iron et al. 2004; Lee 1994) (for a detailed discussion, see Chap. 9). It has been estimated that in the USA alone, there are up to four million adults with chronic back pain who have failed conservative therapy (Masuda and Lotz 2010) and although there are a number of surgical options for these individuals, they all have limitations (Chou et al. 2009; Kishen and Diwan 2010; Raj 2008). Discectomy which relieves pain (325,000 operations performed in 2004 in the USA) (2008) does not restore disc height or its original load-bearing capacity (Putzier et al. 2005). Spinal fusion (375,000 surgeries in 2004 in the USA) (2008) is another commonly performed treatment; as has been discussed earlier, this is not always successful, often leading to pseudoarthrosis and limited flexibility (Kishen and Diwan 2010; Mirza and Deyo 2007). Although controversial, some studies suggest that this type of treatment may induce degenerative changes in adjacent vertebrae (Huang et al. 2006; Javedan and Dickman 1999; Kim and Branch 2006; Lee et al. 2012a, b; Schulte et al. 2007). Disc replacement with a motion-preserving prosthesis, such as partial or total disc replacement, is another option (Fekete and Porchet 2010; Kishen and Diwan 2010; Shim et al. 2007; So et al. 2007; Vernengo et al. 2008). However, there are numerous clinical contraindications to this treatment (Fekete and Porchet 2010; Shim et al. 2007), and these replacements do not fully restore kinematics (Fekete and Porchet 2010). This topic is discussed in considerable detail in Chap. 14. As well, disc prostheses can generate wear debris (van Ooij et al. 2007) and the reaction that this incites has the potential to be catastrophic given its location near major vessels and nerves. The long-term exposure to ions, such as cobalt and chromium, which can be detected in the serum after disc replacement is unknown (Zeh et al. 2009). Given all these issues, there has been great interest in developing new therapeutic options such as biological approaches for the treatment of chronic symptomatic disc disease (Bron et al. 2009; Kandel et al. 2008; Masuda and Lotz 2010; O’Halloran and Pandit 2007; Richardson et al. 2007). Cell-based therapies that regenerate the disc avoid the functional impact on the disc and/or adjacent tissues as well as the consequences of metal fatigue and the reaction to wear debris (Guyer et al. 2011; Tumialan and Gluf 2011). Importantly the regenerated tissue can remodel and respond to load in a way synthetic prostheses or fused discs cannot.
26.1 Introduction
In an autopsy study, 97 % of individuals 50 years or older had histological evidence of intervertebral disc degeneration, a disease process that involves the annulus fibrosus, nucleus pulposus, and cartilaginous endplate (Miller et al. 1988). The back pain that can develop as a result of this disease has a lifetime prevalence of up to 80 % (Manchikanti et al. 2009; Takatalo et al. 2011, 2012). Approximately 1 in 50 Canadians becomes disabled by back pain which is responsible for 40 % of all workplace absences (Iron et al. 2004; Lee 1994; Rapoport et al. 2004). Although rarely life threatening, the annual total costs in 2002 in the USA as a result of back pain were over $100 billion (Asche et al. 2007; Dagenais et al. 2008; Iron et al. 2004; Lee 1994) (for a detailed discussion, see Chap. 9). It has been estimated that in the USA alone, there are up to four million adults with chronic back pain who have failed conservative therapy (Masuda and Lotz 2010) and although there are a number of surgical options for these individuals, they all have limitations (Chou et al. 2009; Kishen and Diwan 2010; Raj 2008). Discectomy which relieves pain (325,000 operations performed in 2004 in the USA) (2008) does not restore disc height or its original load-bearing capacity (Putzier et al. 2005). Spinal fusion (375,000 surgeries in 2004 in the USA) (2008) is another commonly performed treatment; as has been discussed earlier, this is not always successful, often leading to pseudoarthrosis and limited flexibility (Kishen and Diwan 2010; Mirza and Deyo 2007). Although controversial, some studies suggest that this type of treatment may induce degenerative changes in adjacent vertebrae (Huang et al. 2006; Javedan and Dickman 1999; Kim and Branch 2006; Lee et al. 2012a, b; Schulte et al. 2007). Disc replacement with a motion-preserving prosthesis, such as partial or total disc replacement, is another option (Fekete and Porchet 2010; Kishen and Diwan 2010; Shim et al. 2007; So et al. 2007; Vernengo et al. 2008). However, there are numerous clinical contraindications to this treatment (Fekete and Porchet 2010; Shim et al. 2007), and these replacements do not fully restore kinematics (Fekete and Porchet 2010). This topic is discussed in considerable detail in Chap. 14. As well, disc prostheses can generate wear debris (van Ooij et al. 2007) and the reaction that this incites has the potential to be catastrophic given its location near major vessels and nerves. The long-term exposure to ions, such as cobalt and chromium, which can be detected in the serum after disc replacement is unknown (Zeh et al. 2009). Given all these issues, there has been great interest in developing new therapeutic options such as biological approaches for the treatment of chronic symptomatic disc disease (Bron et al. 2009; Kandel et al. 2008; Masuda and Lotz 2010; O’Halloran and Pandit 2007; Richardson et al. 2007). Cell-based therapies that regenerate the disc avoid the functional impact on the disc and/or adjacent tissues as well as the consequences of metal fatigue and the reaction to wear debris (Guyer et al. 2011; Tumialan and Gluf 2011). Importantly the regenerated tissue can remodel and respond to load in a way synthetic prostheses or fused discs cannot.
26.2 What Is the Goal for Cell-Based Therapies and Tissue Engineering?
The intervertebral disc is a specialized structure consisting of interdependent tissues: the annulus fibrosus which surrounds the centrally placed nucleus pulposus and is integrated into the cartilage endplate (Hukins 1994; Simon 1994). The outer annulus fibrosus is responsible for withstanding circumferential tensile forces while the nucleus pulposus and inner annulus fibrosus resist compressive forces (Hukins 1994; Simon 1994). Together these tissues can handle more load than each tissue alone, stressing the importance of properly integrated structures (Bogduk 1997). The intact cartilage endplate is also necessary. This tissue contributes to the maintenance of nucleus pulposus cell viability, absorbs the water that extrudes from the nucleus pulposus during loading, and prevents protrusion of the nucleus into the adjacent vertebral body (Benneker et al. 2005; Grunhagen et al. 2006; Horner and Urban 2001; Moore 2006; Roberts et al. 1996; Shirazi-Adl et al. 2010). The importance of a properly functioning cartilaginous endplate (Miller et al. 1988) is demonstrated by the fact that animal models of disc degeneration are created by damaging the endplate (Cinotti et al. 2005; Holm et al. 2004) or by endplate calcification (Gruber et al. 2007; Peng et al. 2001; Sahlman et al. 2001). A recent population study showed that the presence of Schmorl’s nodes correlated with disc degeneration and low back pain (Takatalo et al. 2011, 2012). The organization of the intervertebral disc allows the disc to rotate, flex, and resist tensile and shear forces and is critical for proper disc function, especially, as the disc under certain conditions has to withstand up to ten times body weight. Thus, it would appear that for long-term success the goal should be repair and/or replacement of all the damaged tissues of the disc.
26.3 Intervertebral Disc Composition and Architecture: What Will Bioengineered Tissues Have to Recapitulate?
26.3.1 Annulus Fibrosus
While considerable information is provided elsewhere in the book concerning the detailed structure of the annulus, it is worthwhile reminding the reader that the annulus is a very well-engineered tissue and exquisitely adapted to its physiological role. It surrounds the nucleus pulposus and has a cross–ply laminate structure consisting of between 10 and 25 lamellae, each composed of collagen fibers oriented parallel to each other and about 65° from the vertical (Bron et al. 2009; Marchand and Ahmed 1990). The direction of the inclination alternates with each layer such that the fibers in one lamella are 65° to the right, while in the next lamella they are 65° to the left, so every second lamella has the same orientation. Lamella, which can be discontinuous, varies in thickness depending on the location in the disc and ranges from 100 to 600 μm (Marchand and Ahmed 1990). They have a unique insertion into the vertebral body and this differs somewhat between the outer and inner annulus (Nosikova et al. 2012). The collagen concentration is highest in the outer annulus and gradually decreases with progression towards the nucleus (Eyre 1979). The outer annulus fibrosus is composed predominately of collagen I with increasing amounts of collagen II present within the inner annulus. It also contains elastin both within the lamellae and between lamellae (Yu et al. 2002, 2005, 2007), a small amount of proteoglycans of which the most abundant is aggrecan (Inerot and Axelsson 1991) but others such as decorin, biglycan, versican, and fibromodulin are also present (Gotz et al. 1997). The proteoglycans have a differential distribution which is opposite to that of collagen so that the inner annulus fibrosus lamellae are separated by more proteoglycan-rich matrix than those in the outer annulus (Bron et al. 2009). The inner annulus fibrosus merges almost imperceptibly with the nucleus pulposus. The difference in composition between the inner and outer aspects of the annulus fibrosus may reflect the different functions of these regions (Bron et al. 2009; Hsieh and Twomey 2010). Translamellar bridges are seen in the outer annulus and recent work by Elliot et al. suggested that these represent areas of blood vessel regression (Melrose et al. 2008; Schollum et al. 2010; Schollum et al. 2009; Smith and Elliott 2011). It is not known whether they play a role in the biomechanical functioning of the disc. The interface of the annulus fibrosus with the vertebral body is complex, as a portion of the collagen fibers pass through the cartilage endplate into the calcified zone and the remainder sweep laterally to merge with the periosteum (Nosikova et al. 2012).
26.3.2 Nucleus Pulposus
Probably the most important tissue to be engineered in the disc is the nucleus pulposus. It consists of proteoglycans within a loose network of collagen that does not demonstrate the same level of organization as the annulus fibrosus (Bibby et al. 2001; Chan et al. 2011). Proteoglycans comprise up to 65 % of the dry weight of the nucleus. Aggrecan is the major proteoglycan and responsible for binding water thereby enabling the nucleus to resist compressive loads (Chan et al. 2011). Other proteoglycans such as versican, decorin, and fibromodulin are also present (Melrose et al. 2001; Singh et al. 2009; Smith et al. 2009). The nucleus contains predominately collagen II and lesser amounts of collagens III, VI, IX, and XI (Chan et al. 2011). The nucleus contains notochordal cells but the latter cell type either disappears or its morphology changes with age (for a detailed discussion of this topic, see Chaps. 3 and 21) (Choi et al. 2008; McCann et al. 2012; Risbud and Shapiro 2011; Weiler et al. 2010). The notochordal cells likely contribute to disc function and/or maintenance, but how this is achieved is not entirely known at this time (Abbott et al. 2012; Cappello et al. 2006; Risbud et al. 2010).
26.3.3 Cartilage Endplate
The cartilage endplate is a thin (about 1 mm) layer of hyaline cartilage, rich in collagen II. It is integrated with the vertebral body, nucleus pulposus, and annulus fibrosus (Moore 2006). Like articular cartilage, the plate consists predominately of water, proteoglycans, and collagen, but differs from that tissue in organization and amounts of these molecules (Gruber et al. 2007; Moore 2006). Vascular channels penetrate the cartilaginous endplate (Miller et al. 1988), but after growth has ceased, these are obliterated and nutrients and oxygen must diffuse from blood vessels in the vertebral body through the tissue (Benneker et al. 2005; Rajasekaran et al. 2004). In vitro studies suggest that the chondrocytes produce a factor that inhibits TNFα production by nucleus pulposus cells, and thus it may play a role in preventing many of the changes that are characteristic of aging (and degeneration) (Arana et al. 2010). The multiple functions of the cartilage layer in terms of nutrition and oxygen diffusion as well as protection of the nucleus stresses the necessity for an intact cartilage endplate when engineering a substitute tissue.
26.4 The Role of Tissue Architecture in Disc Function
Aside from addressing the needs of the molecular architecture briefly described above, the engineered disc will also need to replace many if not all of the functional requirements of the spine. As a major component of this functional unit, the annulus fibrosus can be viewed as an anisotropic, nonlinear, viscoelastic tissue (Hsieh and Twomey 2010). As discussed in detail in Chaps. 2 and 7, compressive loading causes disc narrowing and outward bulging, placing axial compressive forces as well as tensile (biaxial) strains along the circumference of the annulus fibrosus (Nerurkar et al. 2010a, b). Part of the compressive force is transmitted by the nucleus from one vertebral body to the next, decreasing the load borne by the annulus. Sudden increases in compressive stress are propagated through the vertebral column; because of interpositions of the intervertebral disc, changes in compressive stress are converted into tensile stain towards the outer annulus (Markolf and Morris 1974). This region experiences tensile strain with some compression in the inner aspect; the inner annulus fibrosus is compressed as it resists the circumferential expansion of the nucleus pulposus. With compression, collagen crimp angles change from outer to inner annulus fibrosus, resulting in both depth-dependent and linear region stiffness (Holzapfel et al. 2005). It has also been suggested that inter-collagen fiber shear is important in strain redistribution (Desrochers and Duncan 2010) which may affect nutrient diffusion (Ambard and Cherblanc 2009). These biomechanical events are relevant to the healthy disc; however, with degeneration of the nucleus pulposus, reorientation of the annulus fibrosus is compromised and tensile forces are transferred to the annulus fibrosus (Adams et al. 1996, 2009; Guerin and Elliott 2006). Finite element modeling suggests that strains in the inner annulus increase with degeneration and that with progressive disease this strain is transferred to the vertebral endplate (Schmidt et al. 2009). Thus, intact and properly oriented and interfaced annulus fibrosus lamellae are necessary for proper responses to loading (Adams et al. 2009; Guerin and Elliott 2006). Furthermore, it appears that annulus fibrosus fiber tension not only limits axial rotation (Krismer et al. 1996) but also limits susceptibility to degeneration (Lotz et al. 2008). These biomechanical principles are critical architectural features to be borne in mind in engineering a new intervertebral disc.
26.5 Tissue-Engineering Components
Diseases that afflict articulating joints in the appendicular skeleton involve cartilage and in some settings bone. In the disc, three tissues, the nucleus pulposus, the annulus fibrosus, and the cartilaginous endplate, are all impacted by the disease process (Miller et al. 1988). From this perspective, in order to achieve proper function, all of these tissues may require repair/replacement. Having to recapitulate three tissues, each with very different molecular architecture and function adds a level of complexity to successful biological disc repair.
The strategies for tissue engineering of disc tissues are similar to that of other tissues, namely, to use a combination of cells, scaffolds, growth factors, and/or mechanical signals. The choice is dictated by the tissue(s) under repair. Given that the disc is always loaded, the repair/replacement tissue will likely experience mechanical forces and signals immediately upon implantation.
26.5.1 Cells
One of the major issues limiting the clinical application of biological disc repair/replacement therapies is the identification of a suitable source of cells. Cells could be obtained from a number of tissues, such as the disc itself, cartilage, or bone marrow. It is not yet clear whether the cells used for nucleus pulposus repair must include notochordal cells. In vitro studies have shown that notochord cells produce factors, such as CTGF, which enhances matrix production by nucleus pulposus cells (Erwin 2008; Purmessur et al. 2011). Some investigations indicate that notochordal cells are critical for the maintenance of nucleus tissue (Risbud et al. 2010; Risbud and Shapiro 2011; Shapiro and Risbud 2010). If these cells are required, obtaining sufficient numbers from any of the sources listed above may be problematic, although recent studies in our laboratory suggest that under the appropriate culture conditions, notochord cell numbers in nucleus pulposus tissue can be increased in vitro (Kandel 2011, unpublished data).
Although nucleus pulposus cells would be ideal to use for tissue engineering, they are limited in number and would have to be expanded in culture to get sufficient numbers (Gruber et al. 1997; Maroudas et al. 1975). In the healthy nucleus, the cell density has been reported to be 4,000/mm2; however, it may even been even lower since, with age, a proportion of the cells become senescent (Roberts et al. 2006). Not only do these cells have a slower proliferative rate, they also have an altered phenotype (Le Maitre et al. 2007). A sufficient number of cells may be obtained from herniated disc tissue; this approach may not be straightforward since these cells may be dedifferentiated with limited ability to regenerate tissue in vitro compared to cells obtained from the nucleus pulposus compartment (Hegewald et al. 2011a).
For the reasons stated above, it may be necessary to utilize allogeneic or xenogeneic sources; these cells carry the risk for immune reaction and disease transmission. If cells from the nucleus pulposus are to be used for tissue-engineering purposes, then genetic problems must be accommodated. As discussed in Chap. 10, polymorphism (Trp2 allele) in the COL9A2 gene encoding the alpha2 chain of collagen IX can predispose an individual to disc degeneration (Richardson and Hoyland 2008; Jim et al. 2005). Non-degenerated discs from these individuals were found to be mechanically inferior to non-degenerated normal discs (Aladin et al. 2007). This finding suggests that the use of genetically altered primary cells isolated from an otherwise healthy intervertebral disc may not be appropriate for regeneration of normal tissue. Use of nucleus pulposus cells also may be limited as they have been shown to lose their regenerative capacity with age (Kandel et al. 2007). A recent study demonstrated that matrix retention by nucleus pulposus cells obtained from adolescent cows is impaired and that compared to those obtained from younger animals (calves), matrix gene expression is decreased (Kandel et al. 2007). If similar alterations occur in human cells, it may not be appropriate to use nucleus pulposus cells from older patients unless conditions are identified that “prime” the cells and encourage reversion to a more juvenile geno/phenotype.
Many of the issues limiting the use of differentiated cells may be overcome using autologous stem cells. Developmentally, the annulus cells are derived from mesoderm, whereas nucleus pulposus cells are derived from the notochord (Chan et al. 2011; Choi et al. 2008; McCann et al. 2012). Thus, these cells could be obtained from a number of different tissue types, the most common being bone marrow (marrow stromal cells, MSCs) or adipose tissue (adipose-derived stem cells) (Ahmed et al. 2007; Bieback et al. 2008; Zuk et al. 2002). MSCs have the capability to commit to several different lineages including cartilage, bone, or adipose tissue in vitro, and it is speculated that they can also give rise to disc cells although the evidence for this is limited. Generating nucleus pulposus or annulus fibrosus cells from MSCs has been stymied by our lack of knowledge of tissue phenotype such as the characteristic molecular profile of these cells, a topic considered in detail in Chap. 11.
Despite an ever-increasing number of studies of stem cells and cells of the nucleus pulposus, difficulties still exist in confirming that a stem or stromal cell has differentiated along the disc lineage. For example, Steck et al. suggested that MSCs can adopt a gene expression profile resembling native disc cells but the molecules they examined are also present in the cartilage (Steck et al. 2005). It has been suggested that co-culturing MSC with disc or notochordal cells may be another way to effect conversion to a chondrogenic/discogenic phenotype (Korecki et al. 2010; Le Visage et al. 2006; Purmessur et al. 2011; Richardson et al. 2006; Strassburg et al. 2010; Vadala et al. 2008), possibly via paracrine effects or cell-cell interactions (Vadala et al. 2008). Given that MSC can differentiate to chondrocytes, ensuring that the MSC commit entirely to the nucleus pulposus phenotype has not yet been possible. An alternative approach is to use MSC to secrete factors that activate and stimulate nucleus pulposus cell matrix production and/or accumulation and thus potentiate tissue repair (Doorn et al. 2012; Miyamoto et al. 2010; Strassburg et al. 2010). However, there are further issues associated with the use of MSCs: with age they can lose their regenerative capability (Choumerianou et al. 2010; Erickson et al. 2011). Extensive culture and population doublings may promote senescence, and cell karyotype and gene expression can become altered (Wang et al. 2005; Wilson et al. 2010). For example, a subpopulation of cells in MSC culture was noted to appear morphologically distinct from typical human MSCs (Wang et al. 2005). These cells showed a high level of telomerase activity compared with typical MSCs and formed tumors when transplanted into NOD/SCID mice.
Embryonic stem cells (hESC), another potential source of cells, are derived from the inner cell mass of the embryo (Munoz et al. 2008). ESC can be readily maintained as undifferentiated cells under defined conditions, providing an unlimited supply of pluripotent stem cells. They are capable of differentiating into all three germ layers of the embryo and hence all cell types of the human body. While developing new approaches to use hESC as a source of chondrocytes is an area of intense investigation, little work has been done in this regard with disc cells. To date, deriving chondrocytes from embryonic stem cells that can form articular cartilage tissue has not been fully accomplished, suggesting that identifying conditions that induce differentiation of hESC to disc cells in cell culture is a long way off. Interestingly, in one study mouse ESC were differentiated in vitro to chondrocyte-type cells; these cells transdifferentiated to notochord cells following implantation into rabbit discs (Sheikh et al. 2009). These results suggest that ESC may be a source of notochordal cells, but this topic requires further study. Another issue associated with the use of ESC cells is that they can give rise to teratomas – an unacceptable complication for a treatment of a benign disease (Schriebl et al. 2012). To circumvent this problem, it will be necessary to ensure that there are no residual hESCs after induction of differentiation (Schriebl et al. 2012). Consideration will also have to be given to the ethical concerns related to the collection of these cells which could limit their application clinically.
Inducible progenitor cells (iPSC) hold much promise for disc repair and obviate the ethical issues related to the use of embryonic stem cells. iPSC can be generated by the transfection of the four transcription factors Oct3/4, Sox2, c-Myc, and Klf4 (Yamanaka factors) into somatic cells such as human fibroblasts (Maherali et al. 2008; Takahashi et al. 2007; Takahashi and Yamanaka 2006). The transduced cells return to an undifferentiated, pluripotent state and resemble ES cells (Takahashi et al. 2007). A new technology using virus-independent, transposon-mediated reprogramming of somatic cells into iPS cells has recently been developed (Woltjen et al. 2009), eliminating one of the potential contraindications to the clinical use of these cells. Successful differentiation into cell types such as chondrocytes and melanocytes suggests that iPSC have the potential to be an appropriate cell source for tissue engineering of the disc (Wei et al. 2012; Yang et al. 2011). However, recent studies have identified genetic and methylation changes in iPSCs and issues related to immunogenicity, all of which raise questions as to their suitability for tissue engineering (Barrilleaux and Knoepfler 2011; Lister et al. 2011; Zhao et al. 2011). Clearly, there is much work to be done before these cells can be used in clinical trials (Nakagawa et al. 2008; Yamanaka 2007).
Chondrocytes are another source of cells to use for nucleus pulposus repair. A study by Acosta et al. (2011) showed that juvenile chondrocytes formed cartilage tissue when implanted into porcine discs whereas MSC did not. However, the efficacy of these cells in the long term is not known. As there is increasing evidence that nucleus pulposus cells are different than chondrocytes, even though they produce many of the same molecules, perhaps they should not be used for nucleus repair (Mwale et al. 2004).
There has been little investigation into which cells are suitable to use for annulus fibrosus repair. MSC would seem to be an appropriate source of cells as the annulus fibrosus developmentally arises from the mesoderm. Annulus cells themselves could be used as when grown in monolayer culture, annulus cells maintain their phenotype for up to two passages (Chou et al. 2006). These cells would either have to be obtained from allogeneic or xenogeneic tissues similar to nucleus pulposus cells unless the entire disc is being replaced.
Another potential problem with growing the cells in vitro prior to implantation is their contact with animal products such as fetal calf serum (Tekkatte et al. 2011). Bovine proteins incorporated into the cell membrane could induce an immune response. There is also the risk of infection following implantation (Harrison et al. 2000). Interest in developing alternatives to animal serum to avoid these problems, such as growing cells in autologous serum (Harrison et al. 2000; Lange et al. 2007; Shahdadfar et al. 2005; Tekkatte et al. 2011) or human platelet plasma, is being investigated (Bieback et al. 2009; Schallmoser et al. 2007).
26.5.2 Scaffolds
For disc tissue engineering, roles for scaffolds include retention of cells in a desired location, provision of mechanical properties (sufficient for weight bearing while tissue is growing and maturing), and/or delivery of biochemical cues to the tissue as it is developing or to guide tissue ingrowth (Ikada 2006). Scaffold requirements are such that the material must be biocompatible and biodegradable at a rate that mirrors tissue regeneration. For the nucleus pulposus, a liquid-based scaffold would likely be preferable, whereas for the annulus fibrosus, a fiber-type scaffold would be better. The choice of scaffold is critical as it can affect the tissue that develops. Scaffold fiber diameter and stiffness have been shown to influence cell phenotype, function, proliferation, and orientation (Hadjipanayi et al. 2009; Hsia et al. 2011; Saino et al. 2011). These responses could be utilized in the design of disc scaffolds that enhance cell differentiation.
Adhesion molecules used to coat the scaffolds can also affect cell function (Attia et al. 2010). For example, as shown by Attia et al. (2010), polyurethane scaffolds coated with fibronectin result in an annulus fibrosus-like structure, cells that are aligned and elongated similar to native tissue, and importantly the newly synthesized collagen is also properly oriented parallel to the cell. In contrast if the scaffold is coated with collagen I, the cells assume a polygonal shape and collagen production is delayed and poorly oriented. In addition, the scaffold can influence the recipient tissue reaction after implantation. For example, breakdown products could induce fibrosis or lower the local pH; this is seen when polylactic/glycolic acid scaffolds degrade and release acid which negatively affects cell matrix synthesis (Razaq et al. 2003). One way to overcome some of the problems linked to cell source is to functionalize acellular scaffolds with biomolecules or genes. For example, in a study of intervertebral disc degeneration in rabbits, injection of platelet-rich plasma (containing growth factors) encapsulated in gelatin microspheres into the nucleus pulposus slowed down the disease process compared to untreated animals (Nagae et al. 2007).
26.5.3 Growth Factors
The role of growth factors in maintaining the disc phenotype during cell expansion in vitro and for tissue formation has not been studied extensively. Several studies have shown that growth factors, for example, OP-1 (BMP-7) (Masuda and Lotz 2010), enhance matrix production by nucleus pulposus and annulus fibrosus cells. Treatment of MSCs with growth factors may influence differentiation: one example is TGFβ3 treatment of MSCs maintained on a photo-cross-linked carboxymethyl cellulose hydrogel scaffold (Gupta et al. 2011). Whether the treated cells commit to a nucleus pulposus cell lineage or chondrocyte phenotype has yet to be determined. It has been shown that treatment with a growth factor, such as GDF5, or transduction with growth factor genes can enhance disc repair in vivo. These results lend strong support to the notion that growth factors have a major role to play in biological repair (Masuda 2008; Zhang et al. 2008). Further discussion of this topic is presented in Chaps. 23, 24, and 25.
26.6 Tissue-Engineering Approaches
26.6.1 Nucleus Pulposus Tissue Engineering
Repair of the nucleus pulposus by tissue engineering has received more attention than the annulus fibrosus for two reasons: the nucleus is a relatively less complex tissue and early disc degeneration can occur in the nucleus before being evident in the annulus. A number of different approaches have been taken to engineer the nucleus pulposus such as injections of cells (nucleus pulposus cells, chondrocytes, or mesenchymal stromal cells or stem cells) alone or in a scaffold or implantation of nucleus pulposus tissue formed in vitro prior to implantation.
26.6.1.1 Cell Therapy
A strategy for early disease repair is to inject cells into the disc at a time when only the nucleus pulposus is damaged and the annulus fibrosus and cartilaginous endplate (Miller et al. 1988) are intact. The implanted cells are postulated to work by one of two ways: either by synthesizing matrix or stimulating the endogenous remaining nucleus pulposus cells to synthesize matrix. There is increasing evidence that cell therapy for nucleus pulposus repair may be an appropriate approach. Injection of autologous nucleus pulposus cells or stem cells into the disc has been shown to delay degeneration in both small and large (dog and monkey) animal models (Allon et al. 2010; Crevensten et al. 2004; Ganey et al. 2003; Gruber et al. 2002; Hiyama et al. 2008; Meisel et al. 2007; Okuma et al. 2000; Sakai et al. 2005; Sheikh et al. 2009; Sobajima et al. 2008). In a rat study, the injection of a pellet of both nucleus pulposus cells and MSC was shown to preserve disc height, suggesting that the use of both cell types maybe advantageous (Allon et al. 2010). The duration of these individual studies was variable; the longest was a dog study in which the implant was assessed at 1 year (Ganey et al. 2003). Although the cells used in these trials were for the most part autologous, there were at least two studies that used allogeneic or xenogeneic cells. One was a rat study in which allogeneic MSC were used to repair the disc. There was no evidence of an immune reaction at 1 month, raising the possibility that the disc does provide an “immunoprivileged” environment and that it may be possible to use allogeneic or xenogeneic cells in this setting (Crevensten et al. 2004). In keeping with this premise, mouse ESC which were partially differentiated in vitro to chondrocytes were injected into rabbit degenerated discs: at 8 weeks no immune reaction was observed (Sheikh et al. 2009). Cell labeling studies confirmed that the injected cells remained in the disc. Since both of these studies were short term, longer-term studies are required.
Human cell therapy studies are ongoing. In one clinical trial cervical discs were injected with nucleus pulposus cells harvested from herniated disc tissue. While the 2-year results were promising (Hohaus et al. 2008), the longer-term outcome has yet to be reported. More recently, a pilot study was performed in which ten patients received intra-discal injections of MSCs; there was significant pain relief by 3 months and at 12 months there was increased water content in the disc even though disc height was not fully restored (Orozco et al. 2011).
Another approach to cellular repair is to inject MSC, not into the disc, but intravascularly (Alini M, personal communication 2012). It is expected that the cells would then home to the disc obviating the need for a delivery system or annulus fibrosus needle puncture, which has been shown in animals to alter disc mechanics and in humans to possibly promote disc degeneration (Carragee et al. 2009; Iatridis et al. 2009; Michalek et al. 2010; Zhang et al. 2009). Validation of this approach is lacking at this time, but an in vitro study showing that MSC can migrate into the disc is promising (Illien-Junger et al. 2012).
26.6.1.2 Cell-Seeded Scaffolds
A large number of scaffolds have been developed for nucleus pulposus repair and an overview of these have been published (see reviews) (O’Halloran and Pandit 2007; Yang and Li 2009). In summary, most have been shown to support cell growth and, in some cases, tissue formation. Agarose and alginate have been utilized, but the inability of agarose to degrade and the impurities in alginate may limit clinical application (Bron et al. 2011; Chou and Nicoll 2009). Other examples of scaffolds that have been developed for this purpose include collagen I, atelocollagen type II, collagen II enriched with hyaluronan and cross-linked with polyethylene glycol (4S-StarPEG), polylactic acid, combined thiol-modified hyaluronan and elastin-like polypeptide, chitosan-glycerophosphate, and collagen II/hyaluronan/chondroitin-6-sulfate copolymer sponge which have been seeded with either nucleus pulposus or MSC cells (Bowles et al. 2010; Collin et al. 2011; Halloran et al. 2008; Hegewald et al. 2011b; Huang et al. 2011; Lee et al. 2012a; Nettles et al. 2010; Richardson et al. 2008; Sakai et al. 2005). Some of these constructs have been evaluated in animal models. For example, nucleus pulposus cells seeded into collagen type II/hyaluronan/chondroitin-6-sulfate copolymer sponge were grown in culture for 1 week and then implanted into rabbit lumbar discs immediately after nucleotomy. At 6 months, the disc height was greater in rabbits that received the cell-seeded scaffold compared to scaffold alone; comparison to the normal unoperated disc was not provided. The implanted cells were present and there was evidence of tissue formation (Huang et al. 2011). However, as rabbit nucleus pulposus cells are predominately notochordal, the relevance of this study to humans is not clear. In another study, MSC were seeded into type I atelocollagen and implanted into rabbit discs 2 weeks after nucleus pulposus tissue aspiration. There was evidence of disc repair as the proteoglycan content returned to 83 % of normal. Cells were shown to be necessary as the scaffold alone resulted in only 13 % of the proteoglycan content of the native disc (Sakai et al. 2005). Studies in larger animals have not been performed. There has been increasing interest in hydrogels as they are injectable, able to swell and retain water, and thus withstand loading (Pereira et al. 2013). The plethora of scaffolds that have been generated would suggest that the ideal scaffold has yet to be identified.
26.6.1.3 Tissue Formation In Vitro
Nucleus pulposus tissue can be formed in the presence or absence of a scaffold in vitro prior to implantation. Supporting this approach, a study in rabbits showed that implantation of nucleus pulposus tissue was more effective in delaying disc degeneration than isolated cells (Nomura et al. 2001). There are several advantages to developing tissue prior to implantation. As the disc is always loaded in vivo, a formed tissue that is able to withstand some degree of loading after implantation is an obvious advantage. In addition, the tissue does not have to form in a harsh environment, e.g., presence of proinflammatory cytokines. Furthermore, this approach facilitates formation of more than one tissue type. For example, the authors have developed an in vitro system to form a portion of the intervertebral disc consisting of nucleus pulposus tissue adherent to a subjacent layer of cartilaginous tissue (representing the endplate), thus recreating the inner disc tissue (Hamilton et al. 2006).
26.6.2 Annulus Fibrosus Tissue Engineering
The annulus fibrosus experiences complex loading patterns, for example, under compression on the side of applied bending, there is both tensile radial and compressive axial strain, whereas the opposite side undergoes tensile axial strains (O’Connell et al. 2011). As the annulus fibrosus experiences residual strain even when unloaded (Michalek et al. 2012), it is not surprising that by midlife, about 50 % of individuals have annular tears (Videman and Nurminen 2004). However, the healing potential is limited and it is usually replaced by a fibrous tissue which is biomechanically inferior and herniation of the implant is not uncommon. Thus, once damaged, in order to restore its complex architecture, the annulus fibrosus requires repair and more likely replacement (Bron et al. 2009). Moreover, given the complex loading patterns of the annulus fibrosus, it is critical that the replacement tissue is engineered to closely match the original. Relevant to this issue, the inability of the annulus fibrosus to regenerate also influences the type of nucleus replacements that can be used.
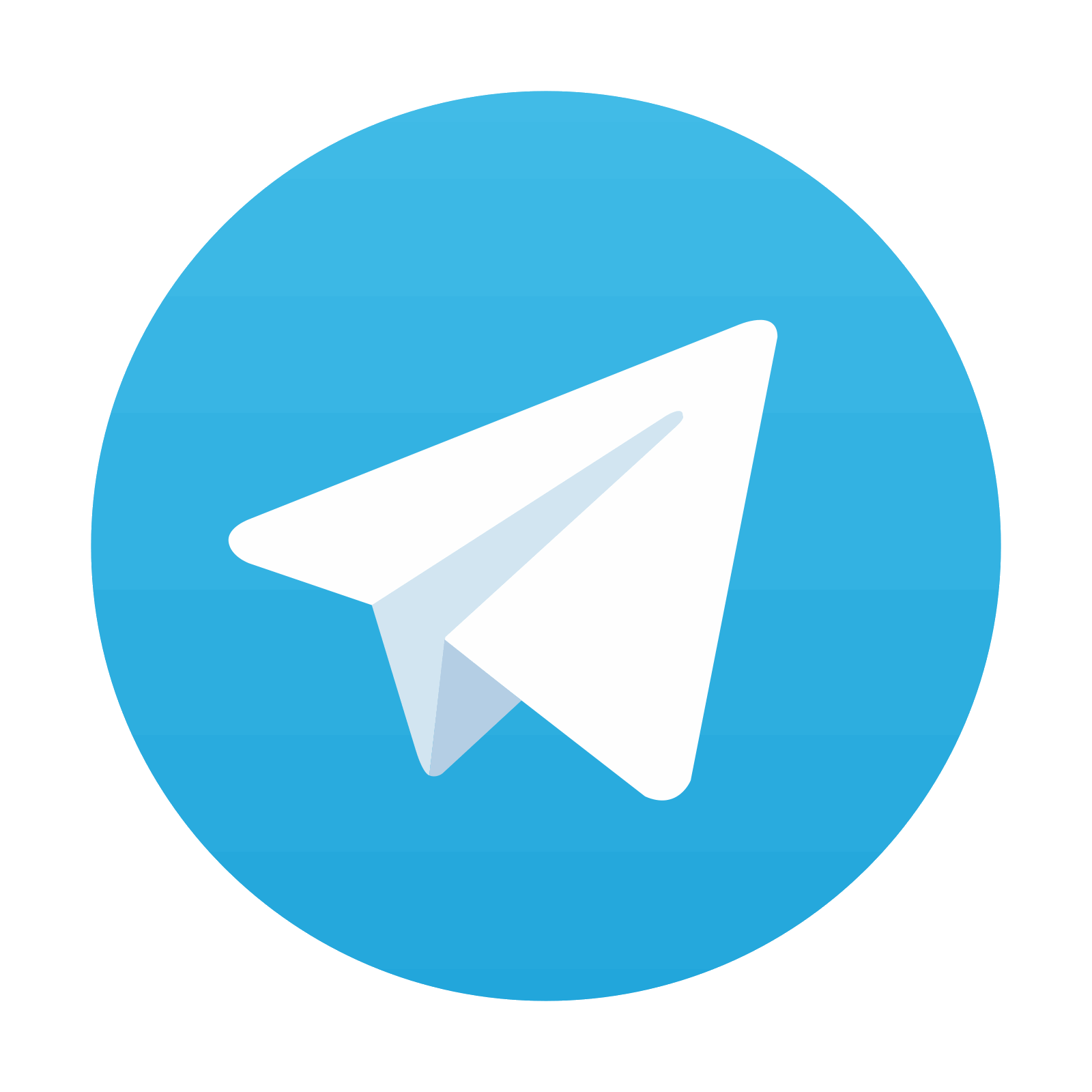
Stay updated, free articles. Join our Telegram channel
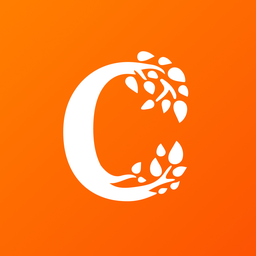
Full access? Get Clinical Tree
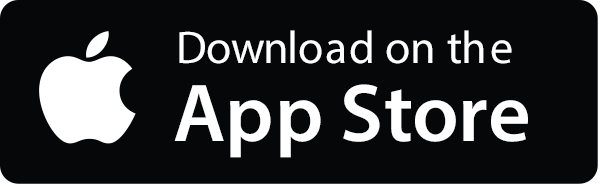
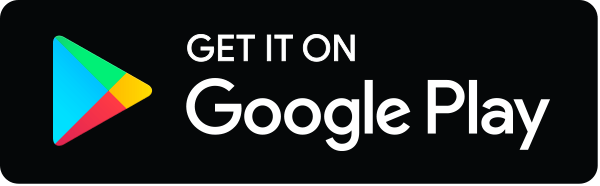