44 Key Points 1. There is evidence for a spinal central pattern generator for locomotion in humans. 2. Human bipedal locomotion uses a quadrupedal limb coordination. 3. A locomotor pattern can be induced and can be trained in complete human SCI when an appropriate afferent input is provided. Locomotion in mammals is largely dependent upon the central pattern generator (CPG); that is to say, neuronal circuits (networks of interneurons) within the spinal cord. The CPG is defined as a neural circuit that can produce self-sustained patterns of behavior, independent of sensory input.1 The understanding of basic principles of CPG function is based on research in invertebrates and primitive fish like the lamprey.2 There is no comparable research in mammals, especially human beings, where our understanding is only based on indirect evidence. Knowledge about the neuronal control of human locomotion is also of broad interest for clinical reasons. Characteristic disorders of locomotion are often the first sign of a central lesion of the motor system. Advances in our understanding of movement control allow us to define more precisely the requirements for the rehabilitation of patients with movement disorders. This chapter focuses on the role of the CPG and its interaction with proprioception during human locomotion. In a more general sense, locomotion is representative of movement control. It is a subconsciously performed, everyday movement that is highly reproducible. It is adapted automatically to existing conditions, such as ground irregularities, within a large safety margin. The selection of, and interaction between, different sources of afferent input is task dependent. Simple stretch reflexes are thought to be involved primarily in the control of focal movements. For more complex motor behaviors, such as locomotion, afferent input related to load and hip-joint position probably has an important role in the proprioceptive contribution to the activation pattern of the leg muscles. There is increasing evidence that the movement disorder after a spinal cord injury (SCI) involves the defective use of afferent input in combination with secondary compensatory processes. This has implications for therapy, which should be directed to take advantage of the plasticity of the central nervous system. For most quadruped mammals, it is assumed that the neural control of locomotion is based on CPGs within the spinal cord.3 This network generates the rhythm and shapes the pattern of bursts of motoneurons.4 For the cat, it is assumed that there is at least one such CPG for each limb and that these CPGs are mainly located within the thoracolumbar region.3 The rhythmogenic capacity of the hindlimb innervating segments of the spinal cord decreases substantially in the rostrocaudal direction, so that the caudal lumbar segments are incapable of producing the rhythm.5,6 Neuronal circuits (networks of interneurons) within the spinal cord that interact with specific sensory information are responsible for locomotion in nonprimate mammals.1 These spinal neuronal circuits are defined as CPGs and were identified as being able to generate self-sustained patterns of locomotor-like neural activity independently of supraspinal and peripheral afferent input.7 Further evidence that neuronal networks in the spinal cord are able to produce rhythmic output was obtained by experiments in which such output was generated even though movement-related afferent input was eliminated through pharmacological blocking of the movements.8 By recording the output at muscle nerves, rhythmic periods of activity, which are reciprocally organized between agonists and antagonists (fictive locomotion), were demonstrated in hindlimbs9 and forelimbs10 of the spinal cat. The CPG model is not restricted to the cat; fictive locomotion has also been demonstrated in a wide variety of invertebrates and vertebrates.11 In contrast to the abundance of data gained from invertebrates, rats, and cats, leading to the general assumption of a CPG underlying the central control of locomotion, there is relatively little known about spinal networks acting like CPGs in humans. Nevertheless, there are observations indicating that the neural control of human locomotion is based on the activity of spinal CPGs.3 The existence of CPGs in humans is difficult to demonstrate because it requires observations of oscillating neural networks after anatomically complete spinal lesions and deafferentation. Nevertheless, several human studies have provided evidence of oscillatory neural networks that interact with afferent input with limited or no detectable functional supraspinal input. Electrical stimulation of flexor reflex afferents show characteristics of neuronal networks in humans12 that are similar to those identified as CPGs in animals. Furthermore, rhythmic contractions of the trunk and lower limb extensor muscles were described in an individual with clinically complete SCI.13 This rhythmic activity could be induced, stopped, and modulated by peripheral stimulation of flexor reflex afferents.14 Also, involuntary steplike movements that were modulated by sensory input were observed in an individual with chronic incomplete cervical SCI several years after injury.15 Pattern generation is basically innate. In humans, steplike movements are present at birth; they are spontaneously initiated or triggered by peripheral stimuli. A central origin of these movements is implied because an electromyographic (EMG) burst precedes the actual mechanical events.16 Central programming can be influenced by sensory input.17 This again is illustrated by infant-stepping. Although rhythmic alternating leg movements are coordinated by a CPG, the infant is unable to maintain body equilibrium. Infants lack an integration of the appropriate afferent input into the programmed leg muscle EMG pattern. To achieve body equilibrium, afferent information from a variety of sources within the visual, vestibular, and proprioceptive systems is utilized by the CPG for adaptation to actual needs (Fig. 44.1). The convergence of spinal reflex pathways and descending pathways on common spinal interneurons seems to play an integrative role18 similar to that in the cat.19 For example, visual feedforward information reduces the activity that arises from the length sensors of muscles (the muscle spindles). Furthermore, the amount of proprioceptive feedback from the legs during various locomotor activities determines the influence of vestibulospinal input on the stabilization of body movement.20 Conversely, somatosensory loss increases vestibulospinal sensitivity.21 The selection of an appropriate locomotor pattern determines the mode of organization of muscle synergies that are designed to meet multiple conditions of stance and gait.22,23 Afferent information influences the central (spinal) pattern and, conversely, the CPG selects the appropriate afferent information according to the external requirements.1,24 Both the CPG and the reflexes that mediate afferent input to the spinal cord are under the control of the brainstem.25 The actual weighting of proprioceptive, vestibular, and visual inputs to the equilibrium control is context dependent and can profoundly modify the central program.23 In addition, proprioceptive information provides the basis for a conscious representation of the body in space, which becomes disturbed in deafferented individuals.26 Furthermore, there is phase-linked corticospinal control of locomotion in humans27 and other mammals.28 Voluntary commands have to interact with the spinal locomotor generator to change, for example, the direction of gait or to avoid an obstacle.29 Spinal reflexes adapt the preprogrammed motor patterns of leg muscles to the terrain.30 Whereas this neuronal mechanism explains quick, unilateral reflex activity in leg extensor muscles, more complex bilateral coordination of leg muscle activation is needed to maintain body equilibrium when gait is disturbed by an obstacle. Irrespective of the conditions under which stance and gait are investigated, the neuronal pattern that is evoked during a particular task is always directed to hold the body’s center of mass over the base of support. Consequently, the selection of afferent input by central mechanisms must correspond to the requirements for body stabilization. Fig. 44.1 Schematic drawing of the neuronal mechanisms involved in human gait. Leg muscles become activated by a programmed pattern that is generated in spinal neuronal circuits (turquoise pathway). This pattern is modulated by multisensory afferent input, which adapts the pattern to meet existing requirements. Both the programmed pattern and the reflex mechanisms are under supraspinal control. In addition, there is differential neuronal control of leg extensor and flexor muscles. Whereas extensors are mainly activated by proprioceptive feedback, the flexors are predominantly under central control.65 The coordination of forelimb and hindlimb rhythmic activities is a main characteristic feature of quadrupedal locomotion.31 Specialized neural circuits located in the caudal spinal cord (the CPG) organize hindlimb locomotor activity, whereas specialized circuits in the rostral spinal cord control forelimb movements.3 The coordination of both circuits is mediated by propriospinal neurons with long axons, which couple the cervical and lumbar enlargements of the spinal cord.32 According to observations made in humans during the last years, bipedal and quadrupedal locomotion share common spinal neuronal control mechanisms. As in quadrupeds, long projecting propriospinal neurons couple the cervical and lumbar enlargements in humans.33 Furthermore, the coordination of limb movements during walking is similar in human infants,34 adults,29 and quadrupeds.1 Nevertheless, there are also distinct differences because the upper limb in primates has become specialized to perform skilled hand movements. The evolution of upright stance and gait, in association with a differentiation of hand movements, represents a basic requirement for human cultural development.35 The phylogenetic development does not, however, exclude that human bipeds still use quadrupedal coordination for their locomotor activities.20 Recent research indicates that interlimb coordination during human locomotion is organized in a similar way to that in the cat.18 Hence, during locomotion, corticospinal excitation of upper limb motoneurons is mediated indirectly, via propriospinal neurons in the cervical spinal cord. This allows a task-dependent neuronal linkage of cervical and thoracolumbar propriospinal circuits controlling leg and arm movements during human locomotor activities (Fig. 44.2). Fig. 44.2 Movement control during different motor tasks. According to the research cited in this review, neuronal control of arm movement is task dependent. (A) During skilled hand movements, strong direct cortical-motoneuronal excitation is predominant (red lines) and the cervical propriospinal neuronal system is inhibited. (B) During locomotion, it is assumed that the brain commands are predominantly mediated by interneurons. Cervical and thoracolumbar propriospinal systems become coupled and coordinate arm and leg movements (red lines). (From Dietz V. Do human bipeds use quadrupedal coordination? Trends in neurosciences 2002;25(9):462– 467. Reprinted with permission.) According to recent studies using functional magnetic resonance imaging,36 the supplementary motor area might be involved in the supraspinal control of this coupling between upper and lower limb movements. There is a neuronal coupling of upper and lower limb muscles during various human locomotor activities.37 In such conditions, arm and leg movements are locked with a fixed frequency relationship. The frequency relationship characterizing this coordination corresponds to that observed in well-defined biological systems consisting of coupled oscillators.38 Also during gait, swinging of the arms serves to regulate the rotation of the body (i.e., it counteracts torsion-related movements of the trunk). These observations indicate a flexible task-dependent neuronal coupling between upper and lower limbs. The pathway that couples upper and lower limb movements seems to become gated by the activity of the CPG during walking. The stronger impact of leg flexors in interlimb coordination is in line with the increasing evidence that leg flexor and leg extensor muscles are differentially controlled in both animals39 and humans.22,27 Evidence for neuronal coupling between upper and lower limbs also comes from studies in patients with cervical SCI. So-called interlimb reflex responses can be evoked with short latency in distal muscles of upper limbs by electrical stimulation of the tibial nerve at the ankle.40 These reflex connections might reflect a loss of supraspinal inhibition or, alternatively, a sprouting of ascending propriospinal systems occupying synaptic locations vacated by degenerating descending connections. Furthermore, it has been shown that the more rostral the spinal cord lesion, the more “normal” the locomotor pattern induced in patients with complete paraplegia or tetraplegia.5 This observation indicates that neuronal circuits underlying locomotor “pattern generation” in humans are not restricted to any specific level of the spinal cord but, rather, that an intricate neuronal network contributing to bipedal locomotion extends from thoracolumbar to cervical levels. There is convincing evidence in spinal animals that use-dependent plasticity of spinal neuronal circuits modifies the sensorimotor function of the adult mammalian lumbosacral spinal cord.41 Regular training after complete spinal cord transection in adult cats improves the recovery of hindlimb function. For example, the lumbosacral spinal cord of the cat could function to execute stepping or standing more successfully if that particular task was specifically practiced. When stand training alone was practiced, stepping ability was compromised.41 Observations in spinal cats also indicated that, if the training of a motor task was discontinued, the performance of that task deteriorated.42 These results show that repetitive motor training provides sufficient stimulation of specific neural pathways to facilitate functional reorganization within the spinal cord and to improve motor output. Furthermore, appropriate sensory input during training is of critical importance to achieve an optimal motor output of the spinal neuronal circuitry.43 Consequently, a much greater level of functional recovery might be possible if the concept of use dependence is applied. The notion that “locomotor-like patterns” can be released also in humans and that there is a basic similarity between the spinal locomotor circuitry of cats and humans is supported by observations made in patients with complete spinal cord transection. In these patients, electrical stimulation of flexor reflex afferents (FRAs) shows characteristics of the neuronal networks similar to those seen in the cat.12,44 Early descriptions of involuntary stepping movements generated by the spinal cord in human subjects with complete paraplegia date back to the work of Lhermitte45 and Kuhn.46 Later on, in a patient with a complete spinal cord lesion, rhythmic contractions of the trunk and lower limb extensor muscles were described.13,47 Furthermore, in persons with complete spinal lesion, spinal cord stimulation is followed by “stepping movements” with reciprocally organized EMG activity of symmetric muscles of both sides.48 Evidence for a spinal CPG for locomotion in humans after SCI has also come from locomotor movements induced on a treadmill with body-weight support in people with incomplete and complete paraplegia.49 Further indirect evidence for the capacity of the spinal CPG has been suggested from recordings of locomotor activity induced in patients with complete paraplegia standing on a moving treadmill with bodyweight support.49–51 According to these experiments, it appears that the strength of the locomotor pattern depends on the level of the lesion (i.e., the higher the lesion, the more “normal” is the pattern).5 This would imply that neuronal circuits up to a cervical level contribute to the locomotor activity, as it was suggested for the mudpuppy.39 The pattern of leg muscle activation during such locomotion resembles in many aspects the pattern observed in an intact cat or a healthy individual. However, the amplitude of leg muscle EMG activity in the individuals with clinically complete SCI is lower than that of healthy subjects, and no independent leg movements resulted from this leg muscle activation.52 After an SCI of the cat, rat, or human, neuronal centers below the level of lesion exhibit plasticity that can be exploited by specific training paradigms. The coordination of human gait seems to be controlled in much the same way as that in other mammals.18 Therefore, it is not surprising that in persons with complete or incomplete paraplegia, locomotor EMG activity can be both elicited and trained, as in the cat. This is achieved by partially unloading (up to 80%) the patients, who are standing on a moving treadmill.29,50,52–54 In severely affected patients, the leg movements usually have to be externally assisted, especially during the transition from stance to swing. It is supposed that by moving the limbs through trajectories under close physiological conditions, providing an appropriate afferent input (i.e., loading, hip joint), spinal neuronal circuits become activated. The timing of the pattern of leg muscle EMG activity recorded in such a condition is similar to that seen in healthy subjects. However, the amplitude of leg muscle EMG is considerably reduced and is less well modulated. This makes the body unloading necessary for the locomotor training. The analysis of the locomotor pattern in completely paraplegic patients indicates that it is unlikely to be due to rhythmic stretches of the leg muscle because leg muscle EMG activity is, as in healthy subjects, equally distributed during muscle lengthening and shortening.50 In addition, recent observations indicate that locomotor movements induced in patients who are completely unloaded do not lead to leg muscle activation.43 This implies that the generation of the leg muscle EMG pattern in these patients is programmed at a spinal level rather than being generated by stretch reflexes. Individuals with an incomplete SCI benefit from locomotor training such that they improve their ability to walk over ground. Load- or hip joint-related afferent input seems to be of crucial importance for both the generation of a locomotor pattern and the effectiveness of the training. However, a critical combination of afferent signals may be needed to generate a locomotor pattern after severe SCI. Several neurotransmitter systems within the spinal cord are suggested to be involved in the generation of locomotor patterns and their adaptation to repetitive use. In spinal cats, serotonin agonists modulate established locomotor patterns, whereas antagonists worsen the locomotor pattern.55 Using a driven gait orthosis (DGO), the effects of locomotor movements restricted to hip joints were studied in people with complete paraplegia.43 An important observation was that the pattern of leg muscle activation was almost unchanged after knee-joint movements were blocked in these patients. Furthermore, isolated joint movements of the foot evoked only local responses. This indicates that afferent input related to hip-joint position also has an important influence on leg muscle activation by the isolated spinal cord. During the course of daily locomotor training, the amplitude of the EMG in the leg extensor muscles increases during the stance phase, and inappropriate leg flexor activity decreases. Such training effects are seen in both complete and incomplete paraplegic patients.50,52 These training effects lead to a greater weight-bearing function of the extensors. This indicates that the isolated human spinal cord has the capacity not only to generate a locomotor pattern but also to show some plasticity. However, only persons with incomplete paraplegia benefit from the training program insofar as they can learn to perform unsupported stepping movements on solid ground.50,52 In complete paraplegic patients, the training effects on leg muscle activation become lost after the training has been stopped.56 There are several reports about the beneficial effect of locomotor training in incomplete paraplegic patients,55,57 and patients who undergo locomotor training have a greater mobility compared with a control group without training.58 Afferent input from receptors signaling contact forces during the stance phase of gait is essential for the activation of spinal locomotor centers43,51 and is important to achieve training effects in paraplegic patients.50,52 Furthermore, hip-joint-related afferent input seems to be essential to generate a locomotor pattern.43 The improvement of locomotor activity could also be attributed to spontaneous recovery of spinal cord function.59 However, recent observations indicate that in both incomplete and complete paraplegic patients, the increase of leg extensor EMG activity occurs independently of the spontaneous recovery of spinal cord function.53,54,59 In severely affected chronic (in-) complete SCI subjects, an exhaustion of leg muscle activity during assisted locomotion was observed.60 It was assumed that a degradation of spinal neuronal circuits deprived of their supraspinal input occurs that could not be reversed by training. This exhaustion of locomotor activity was associated with a decrease of the early component and an increase of the late component of spinal reflex activity.61 The significance of loading for the regulation of stance and gait has previously been established in healthy human subjects.22 Proprioceptive inputs from the extensor muscles, and probably also from mechanoreceptors in the sole of the foot, provide load-related afferent information. The signals arising from the afferent input are likely to be integrated into the polysynaptic spinal reflex pathway, which adapts the programmed locomotor pattern to the actual ground conditions. The role of afferent activity for the rhythmic locomotor pattern is to shape the pattern, control phase-transitions, and reinforce ongoing activity.62 Load-related input has an important role in inducing and training the locomotor pattern in SCI subjects. However, the effects of training are lost over time in people with complete paraplegia.56 These results indicate that there is training-induced plasticity of neuronal centers in the isolated spinal cord that is dependent on specific afferent input. This might be of relevance to future interventional therapies (by combining training with regeneration-inducing agents). When locomotor movements were assisted by a DGO that allows stepping movements to be induced, even with 100% body unloading, physiological locomotor-like movements alone did not lead to leg muscle activation.43 This occurs only in combination with loading of the legs in both healthy subjects and people with complete paraplegia or tetraplegia. The amplitude of muscle activation in the legs is directly related to the level of loading on the legs during stepping of healthy and SCI subjects.51 Therefore, it is not surprising that body unloading and reloading play an essential role in the success of locomotor training in paraplegic patients. However, an appropriate rhythmic loading of one extended leg alone while stepping movements are performed by the contralateral leg is not always sufficient for the activation of the static leg.43 This indicates that a combination of different afferent inputs is required to achieve locomotor-like leg muscle activation. Afferent input from hip joints is important for muscle activation during locomotion in mammals, mainly because it initiates the transition from stance to swing. Hip kinematics have been shown to modulate muscle activation during locomotion in humans.43 The pattern of leg muscle activation was shown to be similar when the knee joint movements are blocked while physiological hip movements are induced. Furthermore, isolated foot joint movements (simulated stepping with or without loading the sole of the foot) evoke only local responses, which is in line with earlier reports.22,63 These results suggest that hip-joint afferents play a role in the leg muscle activation in the functionally isolated human spinal cord. There is increasing evidence that in typical movement disorders, such as spasticity after an incomplete SCI, a defective utilization of afferent input, in combination with secondary compensatory processes, is involved. Furthermore, it becomes evident from cat experiments that neuronal networks underlying the generation of motor patterns are quite flexible after central neural lesions.64 This has implications for therapy. The aim of rehabilitation should be the improvement of function by taking advantage of the plasticity of neuronal centers, and should be less directed to the correction of isolated clinical signs, such as the reflex excitability. To monitor outcome and to assess the effectiveness of any interventional therapy, standardized functional tests should be applied. Locomotor training is an effective method for improving the recovery of walking in many individuals with incomplete SCI. However, at this point, complete recovery of walking is not routinely attained with severe injury. Looking ahead, it may be important to discover combination strategies to further enhance the locomotor output, such as the application of pharmacological interventions, spinal electrical stimulation, and functional electrical stimulation. Furthermore, rehabilitation approaches should be refined and directed to take advantage of the plasticity of the central nervous system and the intrinsic neuronal properties of the human spinal cord.65 However, the most promising approach may be to induce some regeneration of corticospinal axons within the spinal cord.66 In the future, individuals with complete or almost complete SCI may profit from a combination of regeneration approaches and exploitation of neuronal plasticity driven by appropriate retraining of the nervous system, taking advantage of spinal neural networks and critical sensory cues.
The Human Central Pattern Generator and Its Role in Spinal Cord Injury Recovery
Central Pattern Generation
Spinal Locomotor Pattern Generation: Evidence in Animals
Spinal Locomotor Pattern Generation: Evidence in Humans
Interaction between Pattern Generation and Afferent Input
Quadrupedal versus Bipedal Coordination of Locomotion
Central Pattern Generation after a Spinal Cord Injury
Interlimb Coupling after a Spinal Cord Injury
Neuronal Plasticity after a Spinal Cord Injury: Animal Models
Locomotor Capacity of the Isolated Human Spinal Cord
Neuronal Plasticity after a Human Spinal Cord Injury
Significance of Load Receptor Input for Training Effects in Spinal Cord Injury Subjects
Significance of Hip-Joint Afferents for Training Effects in Spinal Cord Injury Subjects
Conclusion
For the exploitation of neuronal plasticity after SCI, the provision of an appropriate afferent input is required.
Hip-joint-related and load receptor afferent inputs are essential to induce locomotor activity and to achieve training effects.
Locomotor activity can be trained in complete paraplegic subjects, without profit for locomotor ability.
In immobilized, severely affected SCI subjects, a neuronal dysfunction develops over time after injury, which limits the success of any regeneration-inducing therapy.
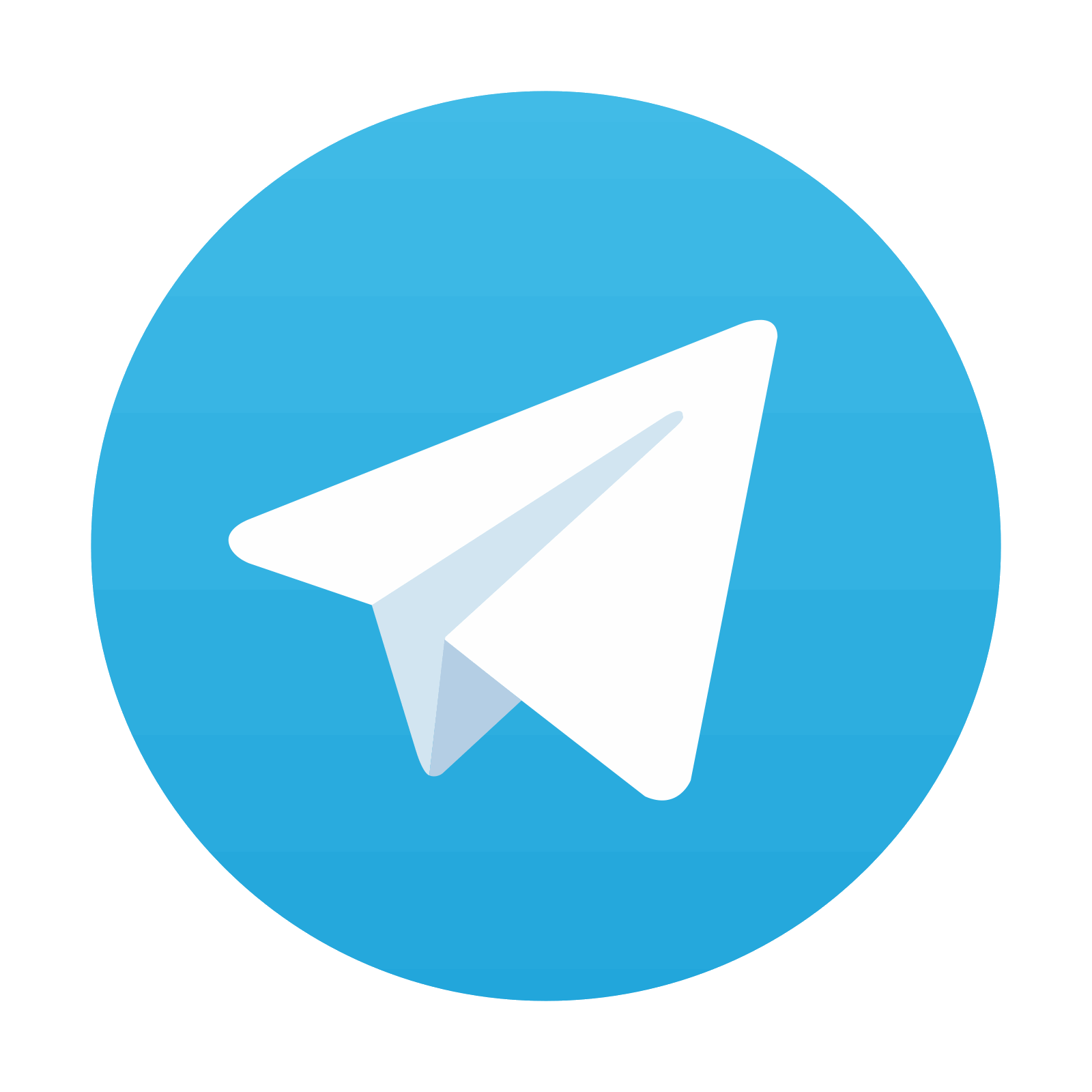
Stay updated, free articles. Join our Telegram channel
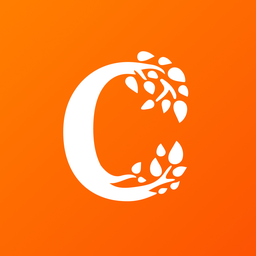
Full access? Get Clinical Tree
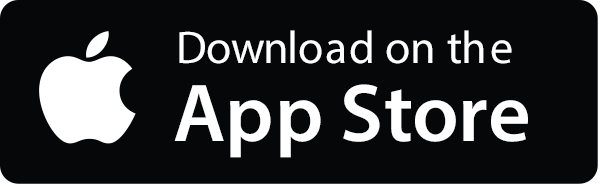
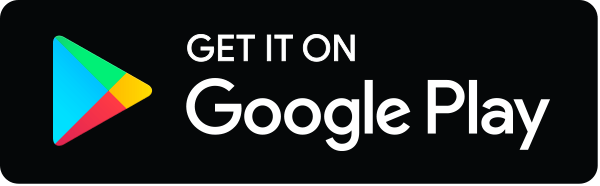