43 Key Points 1. The recovery of motor functions after spinal cord injuries depends on neuroplastic changes occurring at various levels of the central nervous system, including the spinal cord itself. 2. There is abundant evidence in the animal literature of recovery of motor functions like locomotion after complete spinal section. There is also evidence that, after partial spinal lesions, the spinal cord is changed and participates in the recovery of locomotion. 3. There are multiple sites in reflex pathways where transmission can be altered. Spinal lesions may for instance remove important descending inputs that control alternative spinal pathways. 4. Neuroplastic changes may include regeneration of damaged descending pathways as well as changes in various membrane properties of cells in the spinal cord. 5. The evidence of neuroplastic changes as a major component of the recovery of function may justify the use of enhanced sensory inputs to foster the recovery. This chapter discusses various observations and possible mechanisms of neural plasticity following spinal cord injury (SCI). Although plasticity is an underlying theme of several chapters of this book, it is important to integrate some of the neural plastic changes with the known anatomy and physiology as presented in Chapter 1. Although several observations are made in animals, the aim is to highlight some mechanisms (proofs of principle) that should be considered in clinical conditions. It is indeed likely that the underlying mechanisms involved in the recovery of sensorimotor functions are conserved in several animal species, including humans. The expression translational research can imply that concepts developed in one animal species are simply transposed in another species more or less as a physical object is translated in the physical space. In the linguistic sense, translation implies that a concept expressed in one language is expressed in another language using the symbols of that second language. Some of the concepts exposed here must be translated from animals to humans, taking into consideration the specificity of the various species (bipedality, etc.). Although initially well studied in kittens,1,2 the recovery of hindlimb locomotion after complete SCI is just as impressive in the adult cat,3 adult mouse,4 and adult rat,5,6 provided an adequate stimulation is applied (e.g., locomotor training, pharmacology). In cats, a few days after a complete SCI at low thoracic levels, perineal stimulation evokes small alternate movements of the hindlimbs, especially at the hip, on a treadmill belt while the cat stands with its forelimbs on a fixed platform. At this stage the cat inadequately places the paw on the foot dorsum and weight bearing is absent. After 2 to 3 weeks of daily treadmill training (15 to 30 minutes), cats can regain hindlimb walking with some hindquarter weight support while making plantar foot contacts and generating much larger steps. Figure 43.1 compares the hindlimb locomotor pattern of a cat before and 40 days after spinalization. Although there are some differences between the two patterns, the similarities are striking, considering that all connections to and from the brain have been lost. It must then be concluded that the spinal cord can by itself generate the basic locomotor pattern of the hindlimbs. As mentioned in Chapter 1, spinal circuits exist within the cord that are capable of expressing such rhythmicity, even in very primitive vertebrates such as the lamprey.7 The neuronal elements essential for the generation of such locomotor patterns are largely unknown in mammals, but the fact that locomotion can be expressed after a complete SCI is proof of their existence. Among the most prominent deficits in spinal locomotion are the absence of voluntary movements, severe equilibrium deficits, a reduced weight support of the hindquarters, reduced step length, and a variable amount of foot drag at the onset of swing. Diminished weight support probably results from the loss of reticulospinal and vestibulospinal pathways because similar deficits are observed with partial spinal lesions of ventral and ventrolateral spinal pathways. Similarly, the simultaneous activation of hip and ankle flexors may lead to foot drag, which could be seen as the equivalent of a foot drop in humans. Such specific deficits in the timing of hindlimb flexors may result from damage to corticospinal pathways because they are also observed after dorsolateral spinal lesions in the cat.8 The notion of a spinal central pattern generator (CPG) is important in a clinical context because these spinal circuits may contribute to the recovery of locomotion (see Chapters 44 and 45). The existence of a spinal CPG, capable of generating autonomous rhythmic activity in humans, has often been debated. However, there are reports9–11 of patients with complete or incomplete lesions who present involuntary rhythmic movements of the legs (often called myoclonus). In a patient with a complete SCI, it was shown that, despite synchronous discharges in some muscles (even in some antagonists), others may contract out-of-phase,12 as is usually the case between flexors and extensors during locomotion. Such observations should not be too surprising because there are striking descriptions of involuntary movements in the lower limbs of war-injured patients with complete SCI.12 As reviewed in Chapter 12, the lower limbs of some patients with complete spinal thoracic cord lesions had to be strapped to the bed because of uncontrollable large amplitude rhythmic movements involving all the joints of the leg. The importance of the spinal CPG will become even more obvious later, when we give evidence that, in cats, the CPG may have a role in locomotion not only after complete SCI but also after partial spinal lesions. Chapters 44 and 45 provide a more detailed view of this subject. Fig. 43.1 Kinematics and muscle activity in a cat during locomotion before and 40 days after a complete spinal transection. (A) Stick diagrams of swing and stance phases before (black) and after (blue) spinalization in one cat. The stick diagrams are reconstructed from reflective markers positioned over prominent bony landmarks, including the iliac crest, greater trochanter, lateral epicondyle, lateral malleolus, metatarsophalangeal (MTP) joint, and tip of the fourth toe. (B) Angular excursions relative to left foot contact of the hip, knee, ankle, and MTP joints during locomotion. Each line is the average of ∼ 20 locomotor cycles. (C) Single rectified bursts of electromyography obtained with intramuscular wire electrodes during locomotion before and after spinalization in the same cat. Each waveform is the average of ∼ 20 locomotor cycles. St, semitendinosus (knee flexor/hip extensor); Srt, anterior sartorius (hip flexor/knee extensor); VL, vastus lateralis (knee extensor); LG, lateral gastrocnemius (ankle extensor/knee flexor); TA, tibialis anterior (ankle flexor). The cycle time was 1079 msec and 684 msec before and after spinalization, respectively. As discussed in Chapter 1, spinal reflexes are evoked by stimulating peripheral receptors or afferents and by recording the activity within or over a given muscle, which provides a means to evaluate changes in the excitability of sensorimotor pathways following SCI, at rest and during motor behaviors. Reflex excitability is governed by many factors, and it is important to consider all components of the pathway that may be altered after SCI, including peripheral receptors, primary afferents, synapse(s), motoneurons, and properties of the muscle (see Fig. 1.4 in Chapter 1). It does not appear that morphological or functional properties of sensory receptors and axons are greatly affected by SCI.13 On the other hand, once the input reaches the spinal cord, there can be considerable changes in how this input is processed and hence in reflex excitability.14,15 Reflex excitability depends on the baseline activity of the target motoneuron pool, and in the case of di-, tri- and polysynaptic pathways, on the activity of interposed interneurons. It is known that motoneuron excitability is reduced for a time following SCI due to the loss of persistent inward currents (PICs), sustained depolarizing currents that greatly amplify the excitability of motoneurons to a given input.16 Motoneuron excitability and PICs are largely regulated by descending monoaminergic pathways from the brain stem, which are disrupted or completely abolished after SCI. It is probable that interneuronal activity is also depressed due to a similar phenomenon. As a result, spinal reflex excitability is diminished for some time after SCI. Over time, however, PICs return, and as motoneuronal excitability recovers, spinal reflexes return. However, because descending pathways are important in turning off PICs, some reflexes become exaggerated due to abnormal control of neuronal excitability. In other words, a given input produces large sustained responses because the excitability within the spinal circuitry is not as tightly regulated as before SCI.16 Due in part to changes in motoneuron excitability, the muscle can also undergo considerable alterations following SCI in humans and in animal models.17 For instance, after SCI, hindlimb muscle fibers can substantially atrophy and there can be fiber type transformations over time. Changes in the size, number, and composition of muscle fibers can, in turn, influence not only the biomechanical responses of muscles but also the signal recorded over or within the muscle (i.e., electromyography), which can affect reflex excitability measurements. The excitability within spinal reflex pathways is also controlled by direct and indirect interactions between various sources. For instance, supraspinal structures exert a powerful influence on several reflex pathways, as do other reflex pathways at a segmental level18 (see Fig. 1.4 in Chapter 1). Thus it is important to consider how these interactions are modified to gain a better understanding of the pathophysiology of SCI. For instance, some pathways appear much more affected than others after SCI, such as reciprocal inhibition. As described in Chapter 1, in neurologically intact human subjects, stretching a muscle evokes a contraction of the agonist via a monosynaptic excitatory reflex pathway and relaxation of the antagonist via a disynaptic inhibitory reflex pathway (i.e., reciprocal inhibition). However, after SCI, reciprocal inhibition, assessed by conditioning the soleus Hoff-mann reflex (H-reflex) with stimulation of the common peroneal nerve, is reduced in human subjects, and in some cases there is a reciprocal facilitation, instead of inhibition, which produces coactivation of agonists and antagonists at a particular joint.19 Figure 43.2, derived from Fig. 1.4 in Chapter 1, explains how changes in reciprocal interactions can occur after SCI. The coupling between agonists and antagonists, such as ankle dorsiflexors and plantarflexors, is important for the control of movement, and disrupting this coordination can impair motor control. The H-reflex itself is reduced after SCI, but it recovers with time and can be used as a tool to probe the excitability of other pathways, such as presynaptic, recurrent, and reciprocal inhibition, which are also influenced by SCI.14 Fig. 43.2 Appearance of reciprocal facilitation following spinal cord injury (SCI). In humans with SCI, the normal reciprocal inhibition between antagonists mediated by the stretch or H-reflex pathway is disrupted, and instead there can be a reciprocal facilitation producing co-contraction at a given joint.19,56 The figure illustrates the putative activation of an alternate reflex pathway following SCI due to a change in the balance between excitatory and inhibitory connections within the injured spinal cord. In the intact state (left panel), the pathway that mediates reciprocal facilitation is normally inhibited due to mechanisms that are unclear, but after SCI (right panel), the pathway can become active and produce reciprocal facilitation. The dotted lines indicate changes within descending pathways. Cutaneous reflexes, evoked by stimulating the skin or cutaneous nerves, are also modified following SCI.14 For example, a useful clinical tool is the Babinski sign or plantar reflex, which is evoked by slowly passing a blunt instrument on the lateral plantar aspect of the foot.20 After damage to the central nervous system, such as SCI, or in infants in whom the corticospinal tract is immature, the Babinski sign is characterized by dorsiflexion of the big toe and fanning of the other toes. It should be emphasized that SCI most likely does not alter the circuitry of the spinal pathway but produces biases within one of several possible alternate pathways. What is modified is the supraspinal control of this pathway and of other spinal pathways that converge on interneurons interposed in the plantar reflex pathway. Thus the reflex itself after SCI is not pathological, but its control is; a phenomenon that probably extends to most tested reflex pathways. Figure 43.3 explains how a cutaneous pathway may give rise to an inhibitory response or else an excitatory response after SCI. Evaluating changes in reflex pathways after SCI is important because treadmill training in SCI patients is based on the principle that providing sensory cues consistent with walking will induce beneficial plastic changes within the spinal locomotor circuitry.21 Therefore, as the patient “relearns” to walk, activity-dependent processes induce changes within the spinal circuitry and in how it interacts with spared descending pathways and peripheral sensory feedback. Changes in these interactions are thought to be critical in optimizing remnant locomotor functions after SCI. Changes in reflex pathways could also be forerunners for locomotor recovery because some studies have shown that the appearance of long-latency reflex responses, evoked at rest, was associated with the development of walking in humans and adult rats.22,23 These long-latency responses are thought to be components of the spinal locomotor circuitry.11 An alternative explanation is that sensory feedback from the periphery regains the ability to activate key components of the spinal CPG due to the return of excitability within the neuronal locomotor circuitry. Fig. 43.3 Appearance of short-latency excitation after incomplete or complete spinal cord injury (SCI). In the cat, stimulating cutaneous afferents evokes a short-latency inhibition in ankle extensors (E) during the stance phase of locomotion. After complete57 or incomplete SCI, short-latency excitatory responses can be observed following the same afferent stimulation. The most likely explanation is that an alternate reflex pathway becomes activated due to changes within the balance between excitatory and inhibitory connections within the injured spinal cord. The appearance of short-latency excitation can be due to mechanisms acting via the spinal locomotor central pattern generator (CPG) or more directly due to disrupted connections from supraspinal inputs to interneurons interposed in the excitatory cutaneous pathway. The dotted lines indicate changes within descending pathways or from the spinal CPG. As discussed in other chapters, pharmacological interventions may become more and more important in the context of rehabilitation after SCI. The basic approach here may be (1) to replace neurotransmitters, such as noradrenaline and serotonin, that are normally synthesized in the brain stem, which largely disappear after SCI; (2) to offset changes in the excitability of neurotransmitter receptors (i.e., upregulation) caused by the reduction of neurotransmitters; (3) to create a new equilibrium between neurotransmitters released by spared descending inputs and those produced locally in the SC. Pharmacological interventions could be used temporarily to increase the excitability of spinal circuits (i.e., to offset paralysis) or to decrease the excitability of others (i.e., to reduce spasticity), which may interfere with the expression of basic motor patterns.24 The concept of a CPG for locomotion evolved from early work showing that the noradrenaline precursor L-dopa evoked a rhythmic discharge pattern in hindlimb nerves of acute spinal cats paralyzed with curare.25 This “fictive” locomotion resembles normal locomotion with an alternation between flexors and extensors as well as bilateral alternation of both hindlimbs. Noradrenergic agonists, such as clonidine (α-2 noradrenergic receptor agonist), also evoked hindlimb locomotion in spinal cats.26 It is important to mention that the effects of noradrenergic agonists (clonidine) or antagonists (yohimbine) differ whether the cats have an intact spinal cord or a complete or partial SCI. In cats with a large ventral and ventrolateral lesion, intrathecal clonidine injection can altogether stop voluntary quadrupedal locomotion.27 Therefore, the sensitivity of receptors as well as the presence of pre- and postsynaptic receptors in various neural circuits may differ with different spinal lesions, which influences the effectiveness of various drugs. This is an important concept when evaluating a particular drug treatment in humans because the extent of the lesion is hard to quantify and the state of the receptors is completely unknown.28 Although serotoninergic (5-HT) agonists (e.g., quipazine, 5-O-DMT)29 do not initiate locomotion in spinal cats, they strengthen the activity of hindlimb muscles (especially extensors) and paraxial muscles. In cats with ventrolateral spinal lesions, 5-HT agonists increased weight support as well as the ability of cats to walk uninterrupted for prolonged periods.27 5-HT may, however, initiate locomotion in rats when embryonic raphe cells are grafted below a complete SCI5 or when agonists are administered.6 5-HT antagonists, such as cyproheptadine, can also block locomotion or reduce spasticity that may prevent locomotion in humans.30 The importance of better understanding neurochemical changes in the spinal cord after injury is well exemplified by the recent work in spinal rats showing that some serotoninergic receptors can become constitutively active (i.e., their activity then does not require stimulation by an agonist). This could be the basis for the development of spasticity and even be part of the recovery of locomotion.31 Space limitations preclude discussing in detail other neurotransmitter systems, such as amino acids acting on excitatory glutamate receptors or inhibitory receptors acting on gamma-aminobutyric acid (GABA) ergic receptors, which might play a role after SCI. In the latter case, GABAergic agonists like baclofen may be used through intrathecal pumps to relieve spasticity Spinal and Supraspinal Plasticity after Spinal Cord Injury In humans, the majority of SCIs resulting from car accidents or falls are incomplete and may damage several ascending and descending pathways to varying extents. It is therefore important to look at the deficits that result from more circumscribed surgical lesions in animal models as well as other models that approximate human SCI, such as those resulting from spinal compressions produced by clips or weight drops. In cats, none of the spinal quadrants, and therefore none of the descending pathways, plays an indispensable role in the basic generation of locomotion, although severe and specific deficits may be observed as a result of disrupted supraspinal controls.26 Locomotion is generally considered to be initiated via activation of the mesencephalic locomotor region (MLR),32 which activates reticulospinal cells projecting down to the SC and thus the CPG. The reticular formation also plays a major role in the control of locomotion and associated posture.33 Consequently, it would be expected that lesioning reticulospinal pathways would greatly impact locomotion.27,34 Although it is difficult to completely section reticulospinal pathways, the consequences of major damage to these pathways can be observed using ventral and ventrolateral spinal lesions. Cats with such lesions could eventually regain voluntary quadrupedal locomotion but retained diminished weight support and lack of normal coordination between the fore- and hindlimbs. Similarly, humans who had a surgical section of ventral pathways for intractable pain retained walking ability.35 After large lesions of the dorsolateral quadrant,3 cats can walk over ground. Contrary to ventral lesions, after which cats maintained a well organized intralimb walking cycle, cats with dorsolateral lesions had deficits in the temporal recruitment of flexor muscles, leading to a significant foot drag at the initiation of swing, akin to a foot drop in humans. How is this recovery of locomotion achieved? The remaining reticulospinal and rubrospinal cells may participate in the recovery of locomotion (i.e., functional substitution). Propriospinal neurons could be strategically placed to participate in such compensation, as suggested by others.36,37 However, corticospinal pathways may contribute to locomotor recovery, as shown in other chapters. A spinal hemisection implies surgically sectioning half of the spinal cord at a specific segment, and can be grouped into one of three categories (dorsal, ventral, and lateral). In humans, a lateral spinal hemisection is also referred to as the Brown-Séquard syndrome. It is characterized by the loss of sensorimotor functions on the side ipsilateral to the lesion and the loss of temperature sensation on the contralateral side. Brown-Séquard syndrome is most often caused by spinal tumors, piercing trauma (e.g., gunshot or stabbing wounds), ischemia, or vertebral disk herniation. It is a relatively less common form of SCI in humans compared with compressive injuries (see next section). The extent of the lesion and locomotor training are important factors in the recovery of walking following lateral hemisection. In humans, complete paralysis can occur if the secondary injury leads to a large final lesion. In most cases, patients will recover some sensorimotor functions below the injury. In experimental models, a lateral hemisection implies surgically sectioning the left or right side of the spinal cord, which abolishes all ventral and dorsal descending and ascending pathways on one side of the spinal cord. After such lesions in various animal species, treadmill and normal walking recovers within a few days or weeks, depending on the severity of the injury.3 It is very difficult to perform a “perfect” hemisection, but in all cases animals respond well to training regardless of whether the lesion is large or small. Animals with smaller lesions generally recover faster and maintain better locomotor performance over time.38,39 In cats, in the first few days following the hemisection, the limb ipsilateral to the lesion usually exhibits flaccid paresis and drags on the treadmill while the animal adopts a “tripod” gait. Over time the affected limb recovers adequate locomotion with weight support and proper foot placement. Although voluntary quadrupedal locomotion recovers, some deficits can persist over time. For example, large lesions can permanently impair the coupling between the fore- and hindlimbs (i.e., interlimb coordination), with the forelimbs adopting a faster rhythm than the hindlimbs. This might be a strategy to provide more excitability to the hindlimbs via spared propriospinal connections between cervical and lumbosacral CPGs. Lateral hemisections also provide a useful model to evaluate spinal and supraspinal plasticity. For instance, after hemisection, the stance phase of the hindlimb contralateral to the lesion usually occupies a greater percentage of the locomotor cycle in cats,40 which minimizes the amount of time that the affected limb contacts the ground until it recovers sufficient excitability. Over time the asymmetry is reduced or disappears. However, if the hemisection is followed by a complete spinal transection, the asymmetry can reverse, with the stance phase of the ipsilateral hindlimb occupying a greater percentage of the locomotor cycle. More importantly, animals can walk within 24 hours of complete spinal transection, a process normally requiring 2 to 3 weeks of treadmill training. This near immediate expression of locomotion following spinalization indicates that considerable plasticity occurred at the spinal level, whereas the reversal suggests that the return toward symmetry before spinalization was accomplished by supraspinal pathways. Descending motor pathways are also very important for skilled motor tasks, such as reaching/grasping and skilled walking (e.g., horizontal ladder or rope walking), compared with treadmill or normal locomotion. Indeed, marked deficits in reaching and grasping are observed in the ipsilateral forelimb following a lateral spinal hemisection at C5 in adult rats.41 Rats that showed some functional recovery in skilled motor tasks had greater sparing of the corticospinal tract. Moreover, there is evidence that spared descending pathways, such as the reticulospinal tract, are morphologically altered (e.g., collateral sprouting) after lateral hemisection of the spinal cord, and that this plasticity is associated with locomotor recovery.42 Therefore, after lateral spinal hemisection, motor adaptation is a distributed process involving spinal and supraspinal structures. The extent of this plasticity, both functional and morphological, and the relative contribution of specific structures in the recovery of locomotion largely remain to be determined. Experimental compressive lesions are usually made in one of two ways. The first method involves using a calibrated clip to compress the spinal cord for a specific amount of time, whereas the second method involves dropping a weight on the exposed spinal cord from specific heights. In both methods the extent of injury can be graded by changing the amount of time that the clip is applied, or by varying the height of the weight-drop. Contrary to surgical sections of the spinal cord, compressive injuries produce more diffuse damage with a central cavitation surrounded by spared white matter (i.e., the secondary injury). The secondary injury is important because it can account for a substantial proportion of the final lesion. In general, the cavitation volume and lesion extent play a large role in determining locomotor recovery. In addition, following a compressive SCI in adult rats, the extent of spared white matter is more closely associated with locomotor recovery than spared gray matter,43 indicating that the integrity of descending motor and ascending sensory pathways is critical for locomotor adaptation. In the adult rat, the same type of compressive injury at T13–L2 causes more severe locomotor deficits than at L3–4.43 Damage at T13–L2 might damage key elements of the spinal CPG, which in the rat are thought to be located at upper lumbar levels.44 The locomotor deficits and extent of recovery following compressive SCI are comparable to those for other types of lesions. Hindlimb locomotion generally recovers over time, and locomotor training is effective in facilitating the recovery of walking. More severe compressive injuries can permanently disrupt the coordination between the fore- and hindlimbs. Inputs from peripheral afferents also appear to be involved in locomotor recovery following compressive SCI. For instance, following a compressive injury at T9, swimming in adult rats is effective in enhancing normal walking.45 Moreover, providing cutaneous feedback to the feet during swim training further enhances locomotor recovery, as is also found following a lateral spinal hemisection in the chick.46 It is likely that the processes involved in locomotor recovery are very similar in different types of SCI. That is, spared pathways/structures and the extent of injury largely dictate residual sensorimotor functions, whereas the amount/quality of training and pharmacological interventions promote functional recovery by modifying interactions between different control levels of the neuraxis. However, even mild compressive SCIs can completely abolish the corticospinal tract, which can have profound consequences on skilled motor tasks.47,48 On the other hand, rubrospinal and vestibulospinal pathways are only partially damaged. Recovery of motor skills after compressive SCI might be accomplished by functional substitution, where other descending pathways take over the function normally accomplished by other structures. If some corticospinal axons are spared, locomotor training can enhance their effectiveness. For example, using transcranial magnetic stimulation, it was shown that motor-evoked potentials in some leg muscles were greater following several weeks of treadmill training in human SCI subjects.49 Recently, it was shown that treadmill locomotion induced considerable cortical plasticity after complete SCI in neonatal rats.50 Cortical plasticity also occurs after incomplete SCI, and it is possible that spared descending corticospinal axons are more effective in activating spinal neurons. Although animal models of SCI cannot be directly extrapolated to humans with SCI, the framework provided by animal work has been useful in conceptualizing therapeutic interventions in humans. Some reviews are particularly useful in relating animal work and clinical conditions.21,24,51,52 The first question to raise is whether humans also have a CPG. Although there is no direct evidence, several studies indicate that it might be the case. For instance, patients with complete spinal section after a war injury can develop autonomous rhythmic movements of the legs.12 Patients with SCI may also develop such spontaneous movements, and rhythmic movements can be triggered by electrical epidural stimulation.53 Patients with partial spinal cord lesions can walk, albeit with some deficits.54,55 Animal and human experiments suggesting a potential for an involuntary rhythmogenesis at the spinal cord/brain stem level have encouraged the promotion of locomotor training on a treadmill with body weight support, robotics, or muscle stimulation, with remarkable results (see Chapter 45).
Spinal and Supraspinal Plasticity after Spinal Cord Injury
Spinal Plasticity after a Complete Spinal Cord Section
Recovery of Locomotion
Recovery of Reflexes
Pharmacological Intervention to Trigger or Modulate Locomotor Functions
Supraspinal and Spinal Plasticity after Partial Spinal Cord Lesions in Animal Models
Severance of Ventral or Dorsal Tracts
Ventrolateral Pathways
Dorsolateral Pathways
Hemisections
Compressive Lesions
Studies in Humans
The existence of spinal circuits capable of generating a well-organized locomotor pattern (central pattern generator) in several animal species after complete spinal lesions may indicate similar capabilities in humans.
Reflex studies show that physiological and pathophysiological conditions can bias reflexes toward one or the other alternative pathways. These reflex pathways may participate in the recovery of function.
After lesion, plastic changes occur in the spinal cord. These may include changes in circuitry as well as cellular membrane properties.
Pharmacological interventions could promote or decrease these intrinsic changes in the spinal cord.
Studies with partial spinal lesions reveal that the CPG probably also participates in the recovery of locomotor functions after SCI and would justify basic locomotor training after SCI.
Stay updated, free articles. Join our Telegram channel
Full access? Get Clinical Tree
Spinal and Supraspinal Plasticity after Spinal Cord Injury
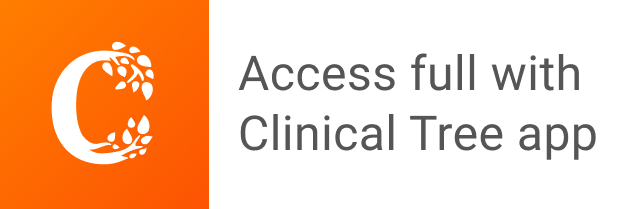