Pharmacology is the broad area of study that deals with how chemical substances affect living tissue on a molecular level and how drugs affect specific patient populations (Table 9-1). Drug therapy is one of the mainstays of modern treatments, and physical therapists often encounter patients who are taking various medications. The Guide to Physical Therapist Practice1 identifies clinical pharmacology as an essential component of appropriate patient monitoring, modality delivery, and communication among medical professionals. It is therefore important for the physical therapist to have a working knowledge of pharmacology because of the number of drugs currently on the market and the number of physical therapy patients that are likely to have been prescribed medications. As physical therapists attempt to account for the effects of their interventions, it becomes apparent that they must also understand the effect and potential interactions of all available and reasonable resources, including pharmacological interventions, offered by other members of the healthcare team.2 The prescription-drug-writing privileges exercised by select military therapists and the evolution of the physical therapy profession promote consideration of an expanded pharmacological role in physical therapy practice.3 Although the dispensing of medications is currently out of the scope of practice for the vast majority of physical therapists, questioning the patient about prescribed medication use can reveal medical conditions that the patient might not consider related to his or her present problem, as the prescribed medications may be for pre-existing conditions that are not directly related to the condition being treated with physical therapy. In addition, this information can also provide the clinician with possible reactions that the patient may have to exercise or other treatment procedures, and the impact on clinical findings. For example: TABLE 9-1 Pharmacology Terms and Definitions Term Definition Drug Any substance that can be used to modify a chemical process or processes in the body, e.g., to treat an illness, relieve a symptom, enhance a performance or ability, or to alter states of mind. The word “drug” is etymologically derived from the Dutch/Low German word “droog,” which means “dry,” since in the past, most drugs were dried plant parts. Pharmacology The science of studying both the mechanisms and the actions of drugs, usually in animal models of disease, to evaluate their potential therapeutic value. Pharmacy The mixing and dispensing of drugs. The monitoring of drug prescriptions for appropriateness and the monitoring of patients for adverse drug interactions. Pharmacotherapeutics The use of chemical agents to prevent, diagnose, and cure disease. Pharmacokinetics The study of how the body absorbs, distributes, metabolizes, and eliminates a drug. Pharmacodynamics The study of the biochemical and physiologic effects of drugs and their mechanisms of action at the cellular or organ level. Pharmacotherapy The treatment of a disease or condition with drugs. Pharmacogenetics The study of how variation in human genes leads to variations in our response to drugs and helps direct therapeutics according to a person’s genotype. Toxicology A study of the negative effects of chemicals on living things, including cells, plants, animals, and humans. Pain medications, muscle relaxants, and nonsteroidal anti-inflammatory drugs (NSAIDs) can mask signs and symptoms, thereby affecting examination findings and increasing the potential for injury during the performance of prescribed exercises.4 However, if the patient has a significant amount of pain, appropriate use of these medications may enhance treatment, allowing a more rapid progression than would otherwise be possible. However, as the patient improves, the need for this medication should lessen. Pharmacokinetics is the study of the physicochemical factors involved as the body absorbs, distributes, metabolizes, and eliminates a drug. To have a desirable effect, a drug must have an appropriate concentration at the site of action. The physicochemical properties of drugs, their dosage formulations, and routes of administration determine the level of drug absorption and the response that occurs. Absorption is the process by which a drug is made available to the body fluids that distribute the drug to the organ systems. The primary routes of administration include oral, buccal, sublingual, rectal, parenteral, topical, and inhalational (Table 9-2). As the target cells become exposed to increasing concentrations of the drug, increasing numbers of receptors become activated, and the magnitude of the response increases until there is a maximal response. In pharmacology, the term bioavailability is used to describe the rate and extent of a therapeutically active drug that reaches the systemic circulation and is available at the site of action. Bioavailability is one of the essential tools in pharmacokinetics, as bioavailability must be considered when calculating dosages. The concept of equivalence among drug products is also important in making clinical decisions: TABLE 9-2 Methods for Drug Administration Method Description Enteral Oral Sublingual or buccal Rectal Chewed, sucked, or swallowed Placed under the tongue or in the cheek Placed in the rectum as a suppository or enema Parenteral Intravenous Intramuscular Intraarticular Subcutaneous Intrathecal Epidural Direct placement of a drug into the bloodstream Injected into the muscle Administered directly into the synovial fluid of a joint Administered below the dermis Injected into the subarachnoid or subdural spaces to bypass the blood–brain barrier Administered into the epidural space Other Inhalational Topical Transdermal Thus, the physicochemical properties of a drug govern its absorptive potential, but the properties of the dosage form (which partly depend on its design and manufacture) and the mode of administration can largely determine drug bioavailability. The volume of distribution is used to indicate how a systemic dose of the medication is ultimately dispersed throughout the body. The volume of the distribution represents how much of the drug appears in the plasma relative to the total amount of drug administered, thus having important implications as to the amount of drug that reaches the target tissue. Because of high levels of blood flow, well-perfused organs such as the heart, liver, kidney, and brain rapidly receive most of the drug during the first few minutes after absorption.6 Tissues with less vascular perfusion such as muscle, skin, and fat require several minutes longer to achieve a steady state. The process of absorption, distribution, biotransformation, and excretion of a drug as related to passage across cell membranes is dependent upon the characteristics of the drug’s molecular size, shape, solubility, site of absorption, degree of ionization, and relative lipid solubility of its ionized and nonionized forms.6 Clearance is the rate at which the active form of the drug is removed or eliminated from the body. It is the time that it takes to clear the drug from blood plasma. The rate of the process to inactivate a drug or eliminate it from the circulation is referred to as the elimination half-life (see later). When administered by most routes (excluding intravenously), a drug must traverse semipermeable cell membranes at several locations before reaching the systemic circulation (Fig. 9-1). These membranes are biologic barriers that selectively inhibit the passage of drug molecules and are composed primarily of a bimolecular lipid matrix, containing mostly cholesterol and phospholipids. The lipids provide stability to the membrane and determine its permeability characteristics. Globular proteins of various sizes and composition are embedded in the matrix; they are involved in transport and function as receptors for cellular regulation. Drugs may cross a biologic barrier by a number of methods: (1) diffusion through the water-filled channels or specialized ion channels, (2) passive diffusion through the lipid membrane, (3) facilitated diffusion, (4) active transport, or (5) pinocytosis. FIGURE 9-1 Drug transfer across a cell membrane. Diffusion. Most drugs are weak organic acids or bases, existing in nonionized and ionized forms in an aqueous environment. The nonionized form is usually lipid soluble and diffuses readily across cell membranes. However, the ionized form cannot penetrate the cell membrane easily because of its low lipid solubility and the high electrical resistance provided by the lipid portion of the membrane. Steroids are nonionized (lipid soluble), the receptors for which are located inside the cell rather than on the external surface of the cell. Distribution of an ionizable drug across a membrane at equilibrium is determined by the drug’s pKa (the pH at which concentrations of nonionized and ionized forms of the drug are equal) and the pH gradient, when present. For a weak acid, the higher the pH, the lower the ratio of nonionized to ionized forms. In plasma (pH, 7.4), the ratio of nonionized to ionized forms for a weak acid (e.g., with a pKa of 4.4) is 1:1,000; in gastric fluid (pH, 1.4), the ratio is reversed (1,000:1). When the weak acid is given orally, the concentration gradient for a nonionized drug between stomach and plasma tends to be large, favoring diffusion through the gastric mucosa. At equilibrium, the concentrations of a nonionized drug in the stomach and in the plasma are equal because only the nonionized drug can penetrate the membranes; the concentration of an ionized drug in the plasma would then be about 1,000 times greater than that in the stomach. For a weak base with a pKa of 4.4, the outcome is reversed. Thus theoretically, weakly acidic drugs (e.g., aspirin) are more readily absorbed from an acid medium (stomach) than are weak bases (e.g., quinidine). Passive Diffusion. In this process, transport across a cell membrane depends on the concentration gradient of the solute. Most drug molecules are transported across a membrane by simple diffusion from a region of high concentration (e.g., gastrointestinal [GI] fluids) to one of low concentration (e.g., blood). Because drug molecules are rapidly removed by the systemic circulation and distributed into a large volume of body fluids and tissues, drug concentration in blood is initially low compared with that at the administration site, producing a large gradient. The diffusion rate is not only directly proportional to the gradient but also depends on the molecule’s lipid solubility, the degree of ionization, and the size and area of the absorptive surface. Because the cell membrane is composed of lipids, lipid-soluble drugs diffuse across the membrane more rapidly than relatively lipid-insoluble drugs. Small molecules tend to penetrate membranes more rapidly than large ones. Facilitated Passive Diffusion. For certain molecules (e.g., glucose), the rate of membrane penetration is greater than predicted due to their low lipid solubility. One theory is that a carrier component combines reversibly with the substrate molecule at the cell membrane exterior, and the carrier–substrate complex diffuses rapidly across the membrane, releasing the substrate at the interior surface. Carrier-mediated diffusion is characterized by selectivity and saturability: The carrier transports only those substrates with a relatively specific molecular configuration, and the process is limited by the availability of carriers. The process does not require energy expenditure, and transport against a concentration gradient does not occur. Active Transport. This process requires energy expenditure by the cell. Substrates may accumulate intracellularly against a concentration gradient. Active transport appears to be limited to drugs structurally similar to endogenous substances. These drugs are usually absorbed from sites in the small intestine. Active transport processes have been identified for various ions, vitamins, sugars, and amino acids. Pinocytosis. This process occurs when a cell engulfs fluid or particles. The cell membrane invaginates (encloses) the fluid or particles, then fuses again, forming a vesicle that later detaches and moves to the cell interior. This mechanism requires energy expenditure and probably plays only a minor role in drug transport, except in the case of protein drugs. The distribution of a drug refers to the movement or transport of a drug to the site of action. Once a drug enters the systemic circulation, it is distributed to different parts of the body including the interstitial and intracellular fluid, and extravascular tissues. The rate at which this occurs depends on a variety of factors including the following:7 Metabolism refers to the process of transforming a drug into a compound that can be excreted. Metabolism occurs primarily in the liver, which reduces the drugs pharmacological activity and lipid solubility. Drug metabolism involves two processes or phases: Drugs are eliminated from the body by a variety of routes including elimination in fluids (urine, breast milk, saliva, tears, and sweat), through the GI tract in the feces, and expelled in exhaled air through the lungs. The kidney is the primary organ for the excretion of drugs that have been inactivated by the liver into water-soluble metabolites.8 Excretion of a drug by the kidneys occurs by two processes7: The rate at which a drug disappears from the body, through metabolism, excretion, or a combination is called the half-life. It is the amount of time required for half of the drug that is in the body to be eliminated. Two terms are used to describe half-life: Knowing the half-life of a drug is critical in determining how often and in what dosage a drug must be administered to achieve and maintain therapeutic levels of concentration. The dosage interval (time between administrations of the drug) is equal to the half-life of a particular drug. The shorter the half-life, the more often the patient must take the medication. Theoretically a steady state will be reached when the amount of the drug taken will equal the amount that is excreted. A steady state is usually reached after five half-lives of the drug have occurred. Thus, a drug with a long half-life may take several days to weeks to reach a steady state. Drug allergies or hypersensitivities range from mild presentations to very severe life-threatening events. For a drug to produce a reaction, it must have antigenic effects and stimulate antibody formation or the formation of sensitized T-lymphocytes, which is immune-related. Drug allergies are generally classified into four types: The effects of exercise on drug distribution are complex and are dependent on factors that pertain to the characteristics of each drug as well as exercise-related factors such as exercise intensity, mode, and duration.10 Exercise increases muscular blood flow and temperature and the subsequent enhancement of absorption through molecular diffusion across biological membranes. The bioavailability of drugs can be altered with exercise primarily because of its influence on the drug’s absorption site.11 For example, there is an increased binding of digoxin (a heart medication that reduces the ventricular rate) in the skeletal muscle.11 On the other hand, exercise may sequester some drugs such as propranolol (a medication used to treat hypertension) in muscle and reduce the availability of the drug for elimination. In addition, exercise decreases the clearance of highly extracted drugs and increases their plasma concentration. Hepatic blood flow has been found to be reduced as much as 50% with an exercise intensity level of 70% of maximal oxygen uptake.12 Decreasing hepatic blood flow could potentially decrease the clearance of drugs that are metabolized as a function of blood through the liver. In a similar fashion, since exercise reduces renal blood flow, the plasma concentrations of those drugs that are primarily eliminated by the kidneys may increase.12 Exercise does appear to enhance absorption from intramuscular, subcutaneous, transdermal (iontophoresis, phonophoresis), and inhalation sites. However, exercise at the site of administration also increases cutaneous blood flow which, in the case of iontophoresis and phonophoresis, could be therapeutically unfavorable as the target site of drug administration could become diluted below therapeutic levels.9 Similar to exercise, physical therapy modalities have the potential to alter the pharmacokinetics of locally and systemically administered drugs, primarily by affecting blood flow and tissue kinetic and metabolic activity.9,13 Thermal agents that increase regional blood flow can theoretically increase delivery of a drug to a specific tissue site although there are a few studies that have documented whether these changes have any clinical relevance. However, application of local heat to the site of the drug administration will almost certainly increase dispersion of the drug away from the delivery site.10 In contrast, cold can theoretically restrict drug delivery by causing vasoconstriction at the cryotherapy site.10 Manual techniques that increase blood flow to an area, such as massage, increase drug absorption from local subcutaneous injection sites. Whether manual techniques have an effect on the delivery of systemically administered drugs is not known at present. In conclusion, because some physical therapy interventions are capable of producing complex changes in the pharmacokinetics of certain drugs, any variation in the clinical response that is observed during or after a physical therapy session, should raise the suspicion about the potential pharmacokinetic effect of a drug.9 In addition, if maintaining the plasma concentration of a drug at a certain level is important, consideration should be given to alternative drugs if the patient is to receive treatment that may affect the absorption, distribution, and metabolism of a drug.13 An understanding of the potential effects of certain types of drugs commonly encountered during the rehabilitation process is essential. Drugs are widely used in the management of both acute and chronic pain and inflammation. By far the most frequently encountered and/or prescribed drug agents in physical therapy practice are those that are prescribed to control pain and/or inflammation. Most of the narcotics used in medicine are referred to as opioids, as they are derived directly from opium or are synthetic opiates.14 Examples of these opioids include codeine, Darvon (propoxyphene hydrochloride), morphine, and Demerol (meperidine) (see “Narcotic Analgesics”). Opioid analgesics are more effective in controlling pain of a constant duration versus sharp intermittent pain.15 Nonopioid analgesics comprise a heterogeneous class of drugs including the salicylates (aspirin and diflunisal), para-aminophenol derivatives (primarily acetaminophen), and the NSAIDs, such as ibuprofen, Motrin, and many others. Despite their diverse structures, nonopioid analgesics have similar therapeutic effects, oral efficacy, and similar side-effect profiles. Nonopioid analgesics are better tolerated compared to opioids by ambulatory patients, have less sedative effects, and are much less likely to produce tolerance or dependence. Conversely, the hazards of long-term administration of these drugs are recognized. Para-Aminophenol Derivatives. Of the para-aminophenol derivatives, only acetaminophen (Tylenol) is widely used. Acetaminophen is not typically classified as an NSAID because of its lack of an anti-inflammatory effect (it does not inhibit prostaglandin synthesis in peripheral tissues). Acetaminophen is primarily centrally acting yet exerts its analgesic and antipyretic effects peripherally by weak inhibition of both isoforms of cyclooxygenase through an unknown mechanism.16 Nonsteroidal Anti-inflammatory Drugs. NSAIDs are distinguished from true steroid agents such as cortisone (cortisol), prednisone (see later), and from the opiate-derived analgesics. NSAIDs have antipyretic, anticlotting (nonselective NSAIDs only), analgesic, and anti-inflammatory effects and are by far the most frequently encountered form of drug in physical therapy practice. NSAIDs are the first-line drug choice for the treatment of mild-to-moderate pain, soft-tissue injury, osteoarthritis, gout, and inflammatory rheumatic disorders.3 Of those patients seeing physical therapists, 25–40% are taking prescription anti-inflammatory agents, with about 40% of those using multiple NSAIDs concomitantly.3,17,18 Aspirin, an acetylsalicylic acid, has been recognized for its pharmaceutical properties for centuries. The pharmacology of aspirin is quite consistent with that of other NSAIDs, and it remains the prototype for comparisons of the efficacy and safety of new medications in its class. Aspirin continues to be the first-line drug for a variety of conditions, including mild pain, fever, osteoarthritis, rheumatoid arthritis, stroke prevention therapy, and potential reduction in prostate cancer incidence.19 Other NSAIDs differ from aspirin in kinetics, duration of action, and patient tolerance, but the overall efficacy is very similar. The analgesic and anti-inflammatory activity of NSAIDs is primarily due to the inhibition of arachidonic acid metabolism.20 Arachidonic acid is present on cell membranes throughout the body and acts as a substrate for prostaglandin, prostacyclin, and thromboxane synthesis.3 Arachidonic acid is released from cell membranes in response to physical, chemical, hormonal, and bacterial or other stimuli.3 NSAIDs also seem to promote the inhibition of the release of cyclooxygenase-1 (COX-1) and cyclooxygenase-2 (COX-2) and the synthesis of prostaglandins at an injury site.20 The pharmacokinetics of NSAIDs have been studied extensively:3 Local Anesthetics. Local anesthetics decrease sensation in a body part without a loss of consciousness or impairments of vital functions that are associated with the use of a general anesthetic.15 All local anesthetics are membrane stabilizing drugs that act by reversibly decreasing the rate of depolarization and repolarization of nociceptor membranes by inhibiting sodium influx through sodium-specific ion channels in the neuronal cell membrane. When the influx of sodium is interrupted, an action potential cannot arise, and signal conduction is inhibited. The local adverse effects of anesthetic agents include neurovascular manifestations such as prolonged anesthesia and paresthesia, which are symptoms of localized nerve impairment. Depending on the local tissue concentrations of local anesthetics, there may be excitatory or depressant effects on the central nervous system (CNS), which can include generalized convulsions, coma, respiratory arrest, and death. Corticosteroids. Corticosteroids can be classified as natural or synthetic. The natural form (mineralocorticoids) is produced by the adrenal glands, under the control of the hypothalamus, and is involved in maintaining fluid and electrolyte balance. Glucocorticoids (cortisol, and drugs such as prednisone), are used primarily for the treatment of a range of immunological and inflammatory musculoskeletal conditions. Although glucocorticoids affect nearly every major organ system in the body, their primary role is to regulate blood glucose. Corticosteroids exert their anti-inflammatory effects by binding to a high-affinity intracellular cytoplasmic receptor present in all human cells,24 which in turn interact with discrete nucleotide sequences to alter gene expression. Because most steroid receptors in target cells are located in the cytoplasm, they need to get into the nucleus to alter gene expression. The side effects from excess corticosteroids are associated with a number of negative side effects, including a catabolic effect on all types of supportive joint tissue. Exogenous steroid use leads to Cushing’s syndrome:25
CHAPTER 9
Pharmacology for the Orthopaedic Physical Therapist
OVERVIEW
Certain medications can produce changes in musculoskeletal structures. For example, prolonged use of corticosteroids may produce osteoporosis and weakening of connective tissues.5
A patient undergoing anticoagulant therapy has a reduced clotting ability and is more susceptible to bruising or hemarthrosis. It is worth remembering that aspirin and aspirin-based products have an anticoagulant effect.
PHARMACOKINETICS
Chemical equivalence refers to drug products that contain the same compound in the same amount.
Bioequivalence refers to chemical equivalents that, when administered to the same person in the same dosage regimen, result in equivalent concentrations of drug in the blood and tissues.
Therapeutic equivalence refers to drug products that, when administered to the same person in the same dosage regimen, provide essentially the same therapeutic effect or toxicity. Bioequivalent products are expected to be therapeutically equivalent.
TRANSPORT ACROSS CELL MEMBRANES
DISTRIBUTION OF DRUGS
The rate of organ blood flow.
The degree of drug ionization in different compartments.
The binding of a percentage of the drug molecules to serum protein. The primary protein that binds drug molecules is serum albumin. Binding prevents the drug from exerting any pharmacologic action. The unbound molecules are the portions of the drug that can penetrate capillary walls to reach the site of action.
The number of competing drugs within the system. Some drugs compete for the same binding sites. This competition may result in higher levels of the unbound drug acting on the body.
Molecular weight.
Blood–brain barrier. Many drugs that easily penetrate other body organs do not appreciably enter the brain because of the sieve-like action of the blood–brain barrier.
Lipid solubility.
Lipid-soluble drugs are more likely to penetrate the blood–brain barrier than other drugs because they pass through the cell membrane.
Lipid-soluble drugs may be stored in adipose tissue, which acts as a drug repository.
Any local metabolism that occurs in any tissue other than the target organ.
METABOLISM OF DRUGS
Phase I. These reactions are catabolic and involve oxidation, reduction, full hydrolysis reactions, with oxidation occurring most frequently.
Phase II. During this phase, the drug undergoes conjugation reactions.
DRUG ELIMINATION
Glomerular filtration, in which drugs are filtered through the glomerulus and then carried through the tubule into the urine. The rate of drug excretion depends upon renal blood flow. Renal blood flow may be diminished with kidney pathology and the aging process.9
Active secretion of the drug by the tubule into the urine.
DRUG HALF-LIFE
Elimination half-life.
The time in which the concentration of the drug in the plasma falls to one-half of its original amount.
A drug’s rate of disappearance from the body, whether by metabolism, excretion, or a combination of both.
Biological half-life.
The time in which the duration of action falls to one-half of its original duration.
The time of the drug’s response rather than its plasma concentration.
DRUG ALLERGY AND DRUG-INDUCED ILLNESSES
Type I (anaphylactic reactions). Anaphylaxis is the most severe allergic reaction and involves the skin and pulmonary and cardiovascular systems, producing cardiovascular and respiratory collapse. The signs and symptoms associated with anaphylactic shock, which usually occur within minutes after antigen exposure, but may still occur up to 1 hour later, include:
Neurological: dizziness, weakness, and seizures.
Ocular: pruritus, lacrimation, edema around the eyes.
Respiratory: nasal congestion, hoarseness, stridor, cough, dyspnea, tachypnea, bronchospasm, and respiratory arrest.
Cardiac: tachycardia, hypotension, arrhythmias, myocardial infarction.
Integumentary: flushing, erythema, urticaria.
GI: nausea, vomiting, and diarrhea.
Type II (cytotoxic reaction). The antigens adhere to the target cell and begin to destroy the target tissue. The clinical manifestations include:
Fever
Arthralgia
Rash
Splenomegaly
Lymph node enlargement
Type III (autoimmune reaction). A complex-mediated hypersensitivity reaction in which the body has difficulty in eliminating antigen–antibody complexes. Manifestations include serum sickness, glomerulonephritis, vasculitis, and pulmonary disorders.
Type IV (cell-mediated hypersensitivity). This type of reaction is mediated through T-lymphocytes as opposed to antibodies. Manifestations include local or tissue reaction.
THE EFFECTS OF EXERCISE ON PHARMACOKINETICS
THE EFFECTS OF PHYSICAL AGENTS ON PHARMACOKINETICS
THE EFFECTS OF MANUAL TECHNIQUES ON PHARMACOKINETICS
PHARMACOTHERAPY
MUSCULOSKELETAL PHARMACOLOGY
Opioid Analgesics
Nonopioid Analgesics
COX-1 inhibitors. COX-1 is constitutively present in virtually all tissues under normal conditions.3 The inhibition of COX-1 by NSAIDs has a tendency to produce a number of adverse effects on multiple organ systems, including GI inflammation, ulceration, bleeding, and increased potential for perforation.21 Other possible side effects include an alteration of normal function in the GI mucosa and kidney blood flow, delayed wound healing, edema, nausea, dyspepsia, and fluid retention. NSAIDs may also alter kidney blood flow by interfering with the synthesis of prostaglandins in the kidney involved in the autoregulation of blood flow and glomerular filtration.22
COX-2 inhibitors. Much attention has been given to the selective COX-2 inhibitors, such as celecoxib (Celebrex), which were developed to provide NSAID benefits without affecting the GI mucosa, renal tissue, or platelet aggregation.3 COX-2 is typically not found on cell membranes under baseline conditions but is induced and upregulated by cytokines, such as interleukin-1 in the presence of cell stress or injury.3 Because COX-2 inhibitors do not produce the same GI effects as COX-1 inhibitors, they are safer to use in patients who are predisposed to gastric or kidney malfunctions. COX-2 drugs block only the COX-2 enzyme, which is responsible for triggering pain and inflammation.20 Because COX-1 is not affected, the patient’s stomach lining is protected, and bleeding tendencies are avoided. However, the enthusiasm for the coxibs have been somewhat tempered by data suggesting they are associated with a higher risk of cardiovascular events than the nonselective NSAIDs, which led to the withdrawal from the market of two widely distributed drugs: Vioxx and Bextra.23
Absorption. NSAIDs are typically lipid-soluble weak acids and are ideally suited for rapid absorption from the acidic stomach and duodenum. After ingestion, most NSAIDs will exhibit an effect within 15–30 minutes. The time interval to perceive symptom relief varies with the rate of enzymatic breakdown of previously synthesized prostaglandins and the time required for the ingested drug agent to inhibit replacement. Once in the bloodstream NSAIDs, except for aspirin, are heavily bound to plasma proteins. Despite the high level of NSAID protein binding, a therapeutic effect is exerted by the constant dissociation of the drug from its binding protein and release as free or active fraction within the serum. Because of NSAIDs’ high-protein affinity they may displace other more weakly protein-bound drugs unintentionally, such as warfarin (Coumadin), sulfonylurea hypoglycemic agents, and methotrexate.
Distribution. NSAIDs are widely distributed throughout most body tissues.
Metabolism. The liver is responsible for the metabolism and bioconversion of NSAIDs. Integration of the NSAID half-life data into the clinician’s therapeutic modality selection allows for maximal drug benefit and a minimization in any adverse impact on therapy. It is worth noting that drugs with longer half-lives are associated with the highest risk for adverse effect. The effect of a given NSAID will vary among individual patients, sometimes leading to a trial of several different agents in an effort to obtain the best therapeutic effect. In clinical practice, 7–10 days are generally long enough to ascertain the effect of a given drug.
Elimination. NSAIDs are eliminated by renal excretion. Alterations in urine pH can affect the level of ionized or nonionized drug residue and, therefore, rates of elimination.
Cutaneous manifestations. Cutaneous manifestations of hypercortisolism include delayed wound healing, acanthosis nigricans (a velvety, thickened, hyperpigmented plaque that usually occurs on the neck or in the axillary region), acne, ecchymosis after minor trauma, hyperpigmentation, hirsutism, petechia, and striae.
Hypokalemia. Hypokalemia (a potentially fatal condition in which the body fails to retain sufficient potassium to maintain health) is a well-recognized side effect of corticosteroid therapy and is probably related to the mineralocorticoid effect of hydrocortisone, prednisone, and prednisolone. Dexamethasone has no mineralocorticoid effect.
Myopathy. There are two recognized forms of corticosteroid-induced myopathy: acute and chronic. Acute myopathy may in part be caused by hypokalemia, although corticosteroids (especially massive dosages) may have a direct effect on skeletal muscle. Both proximal and distal muscle weakness occur acutely, usually with an associated and significant elevation in serum creatinine phosphokinase, which is indicative of focal and diffuse muscle necrosis. In the more chronic form of myopathy, weakness is more insidious in onset and primarily involves the proximal muscle groups.
Hyperglycemia. Hyperglycemia is a condition in which an excessive amount of glucose circulates in the blood plasma. When hyperglycemia is combined with the immunosuppressive effect of corticosteroids, there is a significant increase in the risk for infection.
Neurological impairments. These can include vertigo, headache, convulsions, and benign intracranial hypertension.
Osteoporosis. Corticosteroids inhibit bone formation directly via inhibition of osteoblast differentiation and type I collagen synthesis and indirectly by inhibition of calcium absorption and enhancement of urinary calcium excretion.
Ophthalmologic side effects. Corticosteroids increase the risk of glaucoma by increasing intraocular pressure, regardless of whether administered intranasally, topically, periocularly, or systemically.
Growth suppression. Corticosteroids interfere with bone formation, nitrogen retention, and collagen formation, all of which are necessary for anabolism and growth.
Stay updated, free articles. Join our Telegram channel
Full access? Get Clinical Tree
Pharmacology for the Orthopaedic Physical Therapist
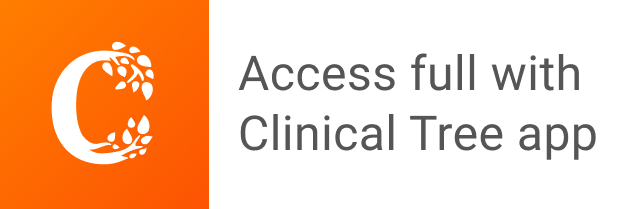