50 Key Points 1. Schwann cell or peripheral nerve grafting can partially counteract secondary injury mechanisms and promote ensheathment and myelination distal to the injury site. 2. Combinatorial strategies have yielded growth of certain critical brain stem tracts into Schwann cell or peripheral nerve grafts with associated improvements in gross locomotor recovery. 3. To date, the required combination of neuroprotective and regenerative strategies has yet to be identified that would promote functional restoration of corticospinal fibers required for fine motor control. Trauma to the adult mammalian spinal cord results in progressive neuronal and glial cell death, axonal degeneration, and functional loss involving motor, sensory, and autonomic systems. Given the limited endogenous repair capacity of the spinal cord, functional recovery can occur either through (1) neuroprotective therapies to reduce secondary tissue loss and associated functional deficits or (2) molecular, cellular, and pharmacological treatments capable of replacing lost tissue or promoting axon regeneration and reconnection to restore lost function. Transplantation of a variety of glial, immune, or undifferentiated stem cells to achieve repair following spinal cord injury (SCI) has resulted in reduced progressive tissue loss1 and has retarded axonal dieback; has promoted sensory, proprio-, and supraspinal axon regeneration2–5; has facilitated myelination3,6,7; and has improved functional outcome.3,4,8,9 Taken together, these studies have demonstrated the versatility of cellular implants in overcoming many key obstacles encountered following experimental SCI, making them ideal therapeutic candidates for repair. Schwann cells (SCs), the myelinating cells of the peripheral nervous system that promote axonal regeneration following peripheral nerve (PN) injury, have also been shown to support central nervous system (CNS) axon growth either as PN grafts or as purified fluid grafts.10–12 SCs provide multiple levels of support for growing axons in vivo; these range from physical guidance for axons by aligning into parallel arrays, to the synthesis of a variety of growth-promoting neurotrophic factors,13,14 and both cell-adhesion molecules, such as N-CAM and L1,15 and extracellular matrix components, including laminin, collagen, and heparan sulfate proteoglycan, which axons attach to and use as permissive substrates for growth and regeneration. Ultimately, SCs ensheathe and myelinate regenerating axons once a stable interrelationship is established. This chapter provides a critical analysis of animal studies investigating the ability of transplanted SCs or PN grafts, alone or in combination, with known neuroprotective and regenerative strategies, to enhance axonal regeneration and functional recovery following experimentally induced contusive, crush, complete transection, or hemisection injuries of the spinal cord. To date, the vast majority of such studies have involved rodents, with assessment of functional recovery performed using standardized measures of simple cortically driven locomotive tasks.16 Although such transplantation paradigms are promising, it remains to be determined whether such grafting will ultimately promote recovery of complex voluntary behaviors, such as reaching and grasping, as seen in humans. In the early 1900s, Jorge Francisco Tello y Muñóz, a disciple of Santiago Ramón y Cajal, was the first investigator to test the ability of PN tissue to promote regeneration in the central nervous system. He placed slices of degenerating PN segments into lesioned rabbit cerebral cortex.17 Histological examination revealed that nascent nerve fibers in the cortex appeared to converge onto the grafted pieces of PN. This finding led Ramón y Cajal to speculate that, given a suitable microenvironment, central neurons indeed could regenerate axons. It was not until the 1980s and the seminal studies performed by Aguayo and colleagues that this initial discovery was ultimately substantiated.10,18 This group showed that, following the removal of a segment of spinal cord and the placement of a piece of PN into the resulting gap, axonal projections were seen to project into the transplanted peripheral nerve. These projections were confirmed by neuroanatomical tracing to originate from the proximal stump central neurons, primarily from adjacent sensory and propriospinal neurons in the spinal cord itself as well as from a subset of brain stem spinal axons.10,19 It thus became established that the PN environment is intrinsically conducive for CNS axon regeneration. Ultimately, this finding led to the observation that SCs are the essential component of this permissive environment,20 which, in turn, laid the foundation for transplantation of SCs into the injured spinal cord. Nevertheless, even at this early stage, Ramón y Cajal recognized that to effectively repair the CNS, a combination strategy involving both support for survival (growth factors) and specific guidance substances would be required.17 Over the past 2 decades, the multiple secondary injury processes that result in progressive destruction of spinal cord tissue (primarily leading to apoptotic cell death of neurons and oligodendrocytes) have been progressively elucidated. In conjunction, research efforts have also progressively focused on combinatorial strategies aimed at counteracting these molecular and cellular obstacles. The development of standardized rodent injury models that recapitulate various aspects of human SCI, along with the established procedures that assess functional recovery, have enabled study of a multitude of potential therapeutic interventions. Human SCI is particularly well modeled by either graded contusion or compression injuries, which allow for varying degrees of sparing depending on the magnitude of the impact used.21–24 For instance, following contusion injuries (which constitute the most common type of SCI reported clinically), both rats25,26 and humans27 form fluid-filled cystic cavities over the course of weeks, resulting in an absence of structural support and axonal growth within the lesion. At the lesion margins, injured axons terminate in dystrophic endings, indicating abortive axonal growth. In this model, an SC-filled polymer tube containing a viscous medium is placed at the rostral stump or between the completely severed stumps of the severed spinal cord.5,12 Given the nature of the injury, the potential of confounding from spared and sprouted fibers is eliminated, allowing identification of regenerated axons with certainty. Axonal conduction across the transected cord has been demonstrated.28,29 Yet, while the primarily sensory and propriospinal axons extending from both spinal cord stumps into the SC bridge are ensheathed and even myelinated (∼ 10%) by SCs, the regenerative response is deficient in several aspects. As when using PN grafts alone,30 these include scar formation at the interface with the spinal cord involving accumulation of chondroitin sulfate proteoglycans,31 very minimal response of brain stem neurons, and the absence of axonal projections across the bridge to enter the spinal cord itself. Because brain stem axons can enter the distal spinal cord when the PN graft lies immediately adjacent to neuronal cell bodies10 (but not when placed remotely within the thoracic spinal cord30), successful axonal regeneration appears to be dependent on the distance between the neuronal cell body and the graft, rather than an inability of brain stem axons to respond to SC cues. Accordingly, several combinatorial paradigms have been evaluated using the complete transection model. These include SCs with methylprednisolone,32 SCs with brain-derived neurotrophic factor (BDNF) and neurotrophin-3 (NT-3) infused around the SC bridge,33 SCs transduced to secrete BDNF implanted into and caudal to the lesion site,34 SCs plus olfactory-ensheathing cells,35 and SCs plus olfactory-ensheathing cells plus chondroitinase.36 As compared with implantation of SCs alone, each of these combination strategies showed a modest but statistically significant improvement in outcome measures, including increased numbers of myelinated axons on the bridge, increased numbers of regenerated axons from brain stem neurons, and increased numbers of regenerated axons extending beyond the bridge into the spinal cord. However, these findings have translated into only modestly improved locomotor function in a few paradigms.36 Alternatively, the use of PN grafts to bridge the interstump gap is associated with several relative potential advantages, including the provision of a suitable microenvironment for myelination of regenerating axons within the SC tubes, the ability to overcome relatively long distances with appropriately sized grafts, and the ability to target axonal growth toward specific target regions of interest. Cheng and colleagues first reported partial restoration of hindlimb function using a paradigm involving a T8–9 laminectomy, removal of a 5 mm spinal cord segment, and implantation of multiple intercostal nerve white matter to gray matter bridges with the engrafted area filled with an acidic fibroblast growth factor (aFGF)-containing fibrin glue37,38 (Fig. 50.1). Detailed gait analysis at 3- and 4-month postinjury intervals revealed progressive improvements in coordinated hindlimb recruitment patterns, with 25 to 30% of movements ultimately involving some hindlimb use in rats treated with both the grafts and aFGF.37 Further investigations employing this approach have confirmed the presence of neurofilament positive axons extending across the grafted area, serotonin-labeled and anterogradely labeled corticospinal axons below the lesion site, as well as several retrogradely labeled supraspinal neuronal populations (including reticulospinal, vestibulospinal, raphe, and red nuclei) whose degree of activation is generally considered to be proportional to the extent of goal-directed locomotive recovery observed following complete spinal cord transection.38–41 In particular, although serotonergic fibers tend to regenerate at a comparatively slower rate, they have been shown to directly activate the spinal locomotor circuit under physiological conditions.42 The PN graft-mediated regeneration is also associated with electrophysiological evidence of both sensory and motor conduction across the transection site.40 Finally, the combined treatment is associated with diminished astrocytic scar formation, activated macrophage migration, and inhibitory proteoglycan deposition,43 all of which are conducive to enhanced CNS regeneration. Fig. 50.1 Reproduction of an original figure by Cheng and colleagues describing their paradigm involving removal of a 5 mm spinal cord segment and implantation of multiple intercostal nerve white matter to gray matter bridges. (From Cheng H, Cao Y, Olson L. Spinal cord repair in adult paraplegic rats: partial restoration of hind limb function. Science 1996;273(5274):510–513. Reprinted with permission. The original PN graft repair strategy reported by Cheng and colleagues38 involved white to gray matter bridging to avoid the presumed inhibition of axonal regeneration by white matter. However, similar aFGF treatment but with direct apposition of the transected spinal cord stumps and placement of surrounding dorsal and ventral PN grafts has also been shown to result in a similar extent of supraspinal axonal regeneration, the presence of lumbar motor potentials, and partial recovery of locomotor function following complete transection.44 Although the intricate bridging of white to gray matter thus does not appear absolutely necessary for functional locomotor recovery, it remains that only modest axonal regeneration is generally observed from supraspinal nuclei; in this study, for instance, functional recovery could only be correlated with corticospinal fiber density in caudal gray matter, which was at least partly due to the overall modest regenerative response achieved. Finally, it remains to be determined whether the observed long-tract regeneration improves functional recovery directly or rather through the stimulation of local neuronal circuitry. Given that regenerating fibers are primarily found within gray matter irrespective of the type of PN graft repair performed, it appears more likely that local interneurons or motoneurons are being stimulated directly to allow central pattern generator–mediated locomotion.44 Following partial spinal cord transections, recovery of function has been associated with plasticity of the corticospinal and rubrospinal tracts rostral to the lesion.45,46 The influence of SC or PN transplants in such injuries is of particular interest given that most human injuries are partial and thus leave spared tissue. As with complete transection, SC implantation also leads to enhanced sensory and propriospinal axon regeneration and myelination.47,48 In fact, axons are observed to extend within the SC hemichannels in rats as early as 2 days postinjury; a few reach the caudal graft–host interface, and, unlike with complete transections, some axons are even found within the caudal cord at 2 or 3 months following injury.48 Although the presence of spared tissue clearly contributes to this enhanced axonal regeneration, as increased fibers are found within Matrigel-only bridges (BD Biosciences, Franklin Lakes, NJ) and some brain stem fibers (e.g., raphe-spinal, coeruleospinal, and rubrospinal) are even seen within the grafts following hemisection injuries,48 the degree of contribution from sprouting of spared fibers remains an unresolved issue. One of the most pressing and as yet unresolved problems in applying the data from these rodent studies to the human condition is to determine whether the observed regeneration is simply influencing the intrinsic and relatively simple central pattern generators in the rodent or whether there is an actual positive influence on more complex behavioral tasks. The testing of voluntary cortically driven tasks in the rodent is limited; thus testing in the nonhuman primate can establish whether grafting will promote recovery of complex voluntary behaviors such as reaching and grasping. Importantly, whether the same regenerative potential as seen with rodents exists in the more complex primate CNS in response to PN grafts or their constituents is not well known. In a study by Levi and colleagues,49 monkeys underwent T11 laminectomies and resection of a 1 cm length of hemispinal cord. In the experimental group, intercostal nerves were grafted along with fibrin glue containing acidic fibroblast growth factor. At 4 months postgrafting, regeneration of the CNS from proximal spinal axons into the PN grafts was observed. One of the basic tenets of regeneration previously established after rodent SCI was thus demonstrated in primates with this study, namely, that regeneration of CNS axons is possible when afforded a permissive environment. However, unlike in rodents, the grafts did not promote any regeneration beyond the lesion site even though an increase in regenerated myelinated axons was observed in the region of the hemisected cord itself. As such, although skilled hindlimb function was not recovered, some improvement in locomotion was noted (as measured by maximal treadmill velocities achieved), perhaps as a result of either trophic influences, local sprouting, or central pattern generator enhancement. The main challenge thus remains to induce the graft-derived regenerating fibers to reenter the injured spinal cord and to establish that the regenerating fibers have a positive influence on more complex (e.g., fine motor) behaviors. SC transplants have been used in numerous rodent studies following moderate to severe thoracic spinal cord contusion injuries. When compared with control rats injected with cell culture medium only, SC-implanted rats contained a ∼ 2.4-fold increase in SC-myelinated axons,11 indicating a contribution beyond that provided by endogenous SCs having migrated into the lesion site. Both transplanted and host SCs survive following contusional injuries, populate and fill the lesional area, and myelinate axons in ratios comparable to those observed following transections.50–53 SC transplants specifically reduce spinal cord cystic cavitation by enhancing neuronal survival and, in most studies, white matter sparing as well, thus accounting for the increased numbers of retrogradely labeled propriospinal and reticulospinal neurons.11,50,53,54 Transplanted SCs also promote axonal growth into contused areas, and, as for transection models, these primarily arise from local propriospinal and sensory neurons with very little penetration from supraspinal axonal populations.11,50,53,54 As such, functional outcome measures, including the Basso, Beattie, and Bresnahan (BBB) rating scale or forelimb and grip strength measurements, have been shown to be only modestly improved in some studies, primarily when white matter sparing was also observed.11,54 White matter preservation thus appears to be a key contribution, because those investigations that failed to demonstrate any improvement in functional outcomes3,50 still demonstrated axonal extension into SC transplants. PN grafts have also been used to promote recovery following acute or chronic spinal contusion injuries.55 In a unilateral cervical contusion model, apposition of a predegenerated tibial nerve to the rostral cyst cavity wall, at 7 or 28 days postinjury, led to enhanced regeneration of brain stem and propriospinal neurons into the PN graft only and not beyond the graft– caudal spinal cord interface. Concomitant aspiration of cellular debris and clot from the cystic cavity reassuringly did not negatively impact on functional outcome measures, indicating that manipulation of the traumatic cyst prior to transplantation may also represent a viable therapeutic adjunct in clinical settings. Nevertheless, in the absence of any additional interventions, no difference in functional recovery is evident in this model beyond that which occurs spontaneously over time.55 Taken together, SC and PN grafts undeniably show multiple beneficial effects following transplantation into the injured spinal cord. They promote white matter sparing and sensory and propriospinal axon growth into the transplanted area. They also myelinate ingrowing axons and help reestablish axonal conduction. However, by themselves they are not sufficient to promote either substantial supraspinal sensory, motor, or autonomic axon in-growth or exiting from the graft area into the host spinal cord. As a result, functional restoration remains lacking, particularly in regard to locomotion. This may be due, in part, to the restriction of SCs to the site of injury and the relative inability to enter and migrate within the astrocyterich environment caudal to the site of the lesion.52,56 The ability to assess SC survival has been limited due to the lack of specific cellular labels for long-term in vivo tracking of transplanted cells. SCs acutely transplanted have nevertheless been shown to have lower survival rates than SCs transplanted 1 week following injury.52 Moreover, SCs appear to fill large cystic cavities only when transplanted together with a synthetic matrix or with a PN graft.36,57,58 Although the degree of white matter preservation has often been assessed in animal studies, it appears that, more specifically, the integrity and identity of the tracts that remain intact (or are restored) are more closely related to functional outcomes.59–61 As detailed later in the chapter, primarily combinatorial strategies to date have yielded growth into the SC grafts of critical brain stem tracts (including reticulospinal, coeruleospinal, and long-descending propriospinal) required for the initiation of stepping movements. In contrast, rubrospinal and especially corticospinal fibers, both of which are more involved with fine motor control, have proven to be poorly responsive to combinatorial strategies used to date.62 Traditionally, SCs have been isolated from PNs and induced to proliferate in vitro to obtain high numbers and pure cell populations. Importantly, similar procedures are available to harvest human SCs, including potentially from the peripheral nerve of a person with SCI. This process is associated with only minor morbidity risks and eliminates the need for the immunosuppression required following autologous transplantation.63–65 Recently, however, several reports have suggested that many of the limitations associated with adult PN-derived SCs may be overcome by obtaining SCs from alternative sources. Cells with an SC-like phenotype have been differentiated in vitro from bone marrow stromal cells (BMSCs) and from skin-derived precursors (SKPs) and examined following transplantation into the completely transected or contused spinal cord.66,67 Both of these non PN-derived SCs were able to enhance the regeneration of descending brain stem axons and also yield modest functional improvements; whereas BMSC-SC bridges enhance non-weight-bearing hindlimb movement in completely transected SCs, SKP-SCs increased the number of animals achieving occasional forelimb–hindlimb coordination by ∼ 46%. SKP-SCs in particular appear promising because, in addition to their ability to populate cystic lesions and myelinate central and peripheral axons, they appear to display improved integration and migration within the normally inhibitory host environment and also to possibly modify adjacent tissues by reducing reactive gliosis and altering growth factor expression.66 Combination treatments with other cell types have been pursued by some investigators to exploit potentially additive or synergistic effects. In particular, olfactory ensheathing cells (OECs) possess certain SC-like properties, but they are also uniquely able to intermingle with astrocytes in vivo and in vitro.68,69 As such, cotransplanted OECs have been shown to enhance the exit of axons from the SC bridge into the caudal spinal cord35 by likely making the SC–astrocyte interface more permissive for axonal crossing. Within the grafted area, OECs appear to play a primarily supportive role because they do not associate with myelinated axonal profiles themselves but rather appear to provide a structural framework for the SCs to, in turn, enhance myelination.70,71 It nevertheless remains controversial to what degree OECs enhance axonal regeneration and functional recovery beyond SC implantation alone,56 with different studies reaching contradictory conclusions with respect to the response of brain stem neuronal populations as well as in myelinated axon counts.1,11 Among several potential confounders, further work will be required to delineate both the optimal source for these cells and the optimal postlesion time for implantation. The majority of SCs undergo necrotic cell death following implantation into either the acute, subacute, or chronically injured spinal cord.51,53 Several pharmacological agents have been shown to reduce post-SCI degeneration, including methylprednisolone, monosialoganglioside, and cyclic adenosine monophosphate (cAMP).72 Interestingly, these agents, in particular cAMP, also appear to independently enhance axonal regeneration.73 Following contusive SCI, immediate and prolonged administration of Rolipram (Sigma Chemicals Co., St. Louis, MO), a phosphodiesterase-4 inhibitor, significantly increases both white matter sparing and the number of SC-myelinated axons within the lesion, and also improves several functional outcome measures.3 When coadministered with SCs and cAMP (triple combination), a significant improvement was noted in the overall BBB score. It remains to be determined whether cAMP directly enhances the survival and myelination capacity of the transplanted cells or whether the recruitment of endogenous SCs is also promoted. Taken together, these cAMP studies, as well as the wealth of data suggesting that methylprednisolone promotes transplant survival and brain stem/propriospinal/sensory axonal regeneration into SC bridges,32,74 suggest that additional neuroprotective agents should be evaluated along with SC transplants. Neurotrophic factors represent strong candidates for combination therapies given their well-recognized ability to promote neuronal survival as well as axonal regeneration/sprouting even on inhibitory substrates. Neurotrophic factor delivery following SCI can promote additional axonal regeneration into SC or PN implants,33 although specific axonal populations respond differentially to specific factors either alone or in combination. Although propriospinal neurons extend projections into SC or PN grafts following glial-derived neurotrophic factor, NT-3, or BDNF factor treatment, sensory neurons appear to be more responsive specifically to nerve growth factor (NGF) and BDNF.7,33,34,75,76 Acidic fibroblast growth factor has been shown to prevent the dieback phenomenon of the corticospinal tract and to promote sprouting and regeneration across the zone of injury through either SC guidance channels or PN grafts following complete thoracic transection injuries.77 Despite the long distances between the delivery site and brain stem neuronal cell bodies, several of these neuronal populations also become responsive when SC or PN grafting is combined with neurotrophic factor treatment.33,34 Finally, neurotrophic factors appear to influence the survival and proliferation of the transplanted SCs themselves because grafts expressing D15A (a chimeric protein that mimics the activity of both BDNF and NT-3) or NGF7,50 are enlarged as compared with controls, and this correlates with enhanced axonal ingrowth. A multitude of extracellular matrix molecules combine with reactive astroglia to form a dense scar in the zone of injury, which in turn acts as a barrier to regenerating axons.78 Thus, additional interventions are necessary to enhance the reentry of regenerating axons into the host spinal cord. One promising approach involves the controlled administration of chondroitinase ABC (ChABC), which has shown efficacy in both SC implantation and PN grafting models.36,79 Following a cervical hemisection injury, ChABC promoted axonal regrowth for ∼ 1.2 mm into the caudal spinal cord, with regenerated fibers showing prominent branching and varicosities at their terminal ends and the extent of regeneration correlating well with functional assessments.79 As expected, exiting axons from an SC-OEC-ChABC graft have been shown to primarily arise from local propriospinal neurons but, interestingly, also from more rostral levels, including raphe, vestibular, and reticular nuclei.36 Given the well-established inhibitory properties of CNS myelin and its constituent proteins for axonal elongation, combination strategies aimed at directly counteracting this effect within the host spinal cord appear particularly attractive80 but have yet to be fully explored. In addition to promoting long-distance regeneration into SC grafts as discussed earlier, BDNF or NT-3 infusion caudal to the SC bridge independently also attracts propriospinal axons beyond the graft and into the host caudal cord tissue over several millimeters.75 Although the underlying mechanisms of this action remain to be fully elucidated, it is possible that these neurotrophic factors support axonal growth over the inhibitory host environment via a cAMP-dependent mechanism.3 Other options include either adjunctive myelin inhibitor blockade (e.g., as for Nogo-A)81 or more general activation of the Rho pathway itself through the use of Rho kinase inhibitors.82 Although SC transplantation and PN grafting remain promising candidates for promoting functional repair following SCI, it is now apparent that they will need to be employed as part of optimized combination strategies. Using SCs from alternate sources or in combination with other cell types, such as OECs, and in conjunction with neurotrophic, glial scar-modifying, or myelin-inhibition blocking factors, may enhance the ingrowth of long-descending axons as well as the exit of fibers caudally into the host spinal cord. Similarly, PN grafts have yet to be assayed using combined strategies to promote regenerating axon graft entry, exit, and subsequent elongation toward synaptic targets. Future investigations will focus on these promising avenues to enhance transplant survival, host tissue sparing, and axonal growth across the implant–host interface.
Peripheral Nerve Grafts and the Repair of Axonal Circuits Following Spinal Cord Injury
Historical Perspective
Grafting in Animal Spinal Cord Injury Models
Complete Transection/Schwann Cell Bridge Models
Partial Transection/Schwann Cell Bridge Models
Contusion/Graft Implantation Model
Remaining Obstacles and Future Prospects 50
Alternative Sources of Schwann Cells
Cotransplantation of Different Cell Types
Neuroprotective and Regenerative Strategies
Promoting Entry and Extension into the Host Spinal Cord
Conclusion
A series of secondary injury processes following SCI combine to promote progressive cell death, degeneration, and functional loss of key fiber tracts. Cellular transplantation has been shown to counteract many of these sources of secondary injury.
SC or peripheral nerve grafting alone results in ensheathment and even myelination distal to the injury site, but the regenerative response is deficient in several respects. This is attributable partly to a poor response of critical brain stem neuronal subpopulations as well as scar formation at the interface with the spinal cord.
Several combinatorial strategies have been shown to further enhance the regenerative response and functional outcome in animal models. These include cotransplantation of different cell types and use of neurotrophic factors, as well as agents that modify the inhibitory extra-cellular environment.
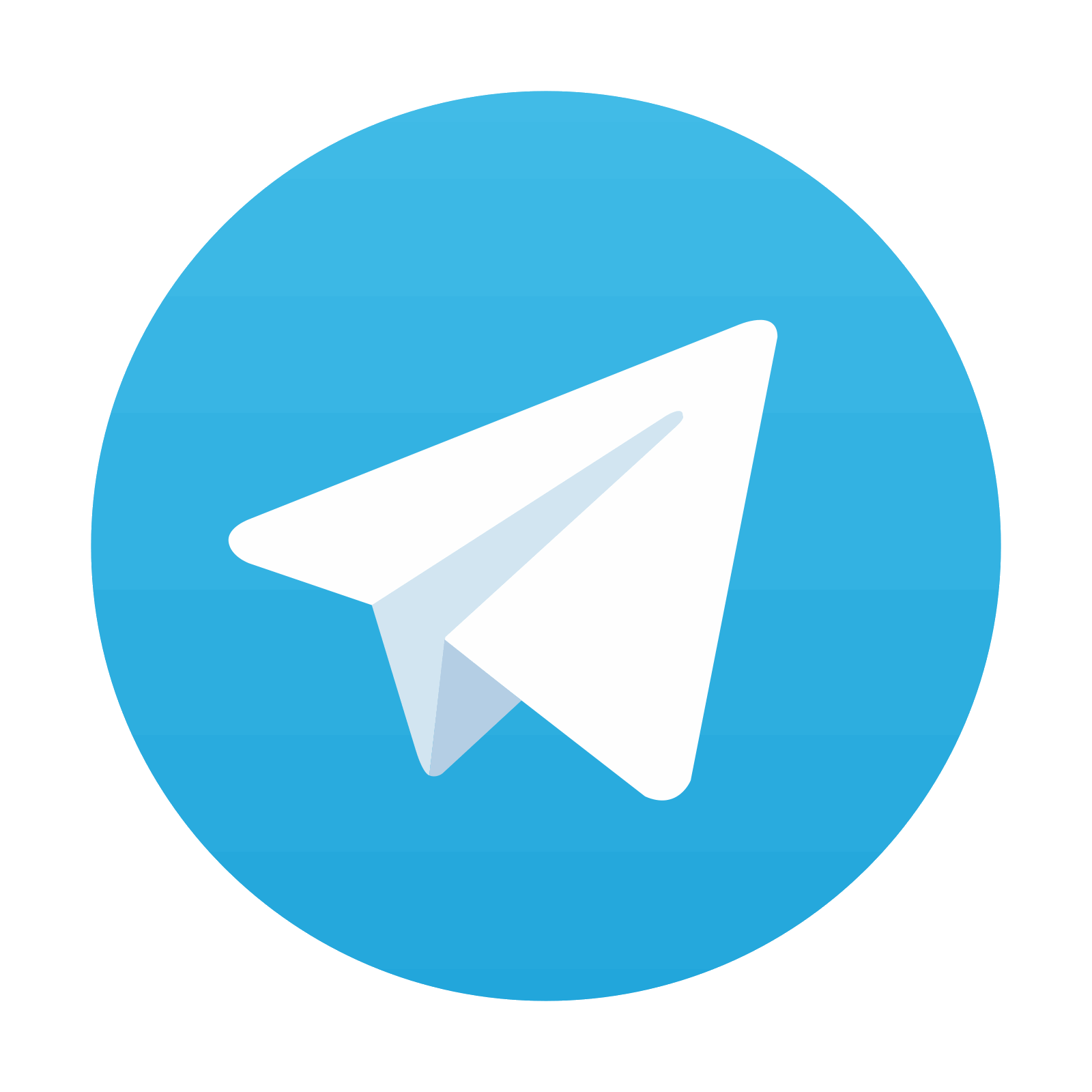
Stay updated, free articles. Join our Telegram channel
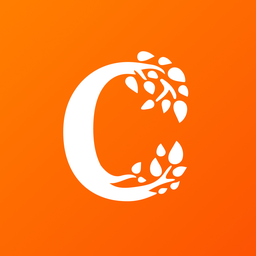
Full access? Get Clinical Tree
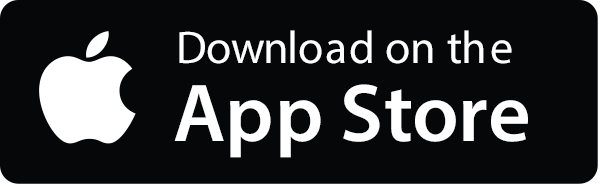
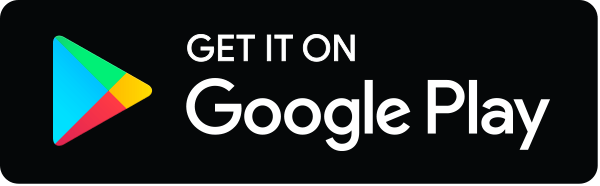