Chapter2 There has been much interest in and reference to stability of the spine over the last few decades. One of the first texts to explore therapeutic exercise for spinal segmental stabilisation in low back pain was published in 1998 (Richardson et al 1998). These authors referred to Panjabi’s model of spinal stabilisation which incorporates a passive subsystem (the osseous and articular structures), the active subsystem (the force generating capacity of muscles which provide mechanical stability) and the neural subsystem (providing control to the muscles) (Panjabi 1992). The concept of stability, movement control and the process of how it is achieved, has different interpretations depending on the background of the authors (Hodges & Cholewicki 2007; McGill 2007). To date there is still debate as to whether spinal stability exists but there is no debate that the spine must be stable to function (Reeves & Cholewicki 2010). This text considers the control of movement and assessment and retraining of uncontrolled movement (UCM) (as described in Chapters 1, 3 and 4) rather than simply a model of stability. All muscles have four broad functions: 1. to concentrically shorten to produce joint range of motion and accelerate body motion segments, which will be termed ‘mobility function’ 2. to isometrically hold position, which will be termed ‘postural control function’ 3. to eccentrically lengthen under tension to decelerate motion and control excessive range of motion, which will be termed ‘stability function’ 4. to provide afferent proprioceptive feedback to the central nervous system (CNS) for coordination and regulation of muscle stiffness and tension. Rood, in Goff (1972), Janda (1996) and Sahrmann (2002) have described and developed functional muscle testing based on stabiliser and mobiliser muscle roles. Table 2.1 describes stabiliser and mobiliser muscle role characteristics. Generating high force may be detrimental in some instances. For example: rectus abdominis is a flexor of the lumbar spine. If it is over-trained and inappropriately strengthened it contributes to pain-related changes in the movement system. It is often over-trained in a misguided belief that it is important to strengthen the abdominals to stabilise and protect the low back or to acquire an abdominal ‘six pack’. However, if this muscle becomes excessively dominant in comparison to the lateral abdominal muscles it increases flexion and compression forces on the lumbar spine and rotation stress and strain is inadequately controlled. This imbalance between rectus abdominis and the lateral abdominal stabilisers has been identified as a common movement-related change in people with low back pain (O’Sullivan et al 1997). 1. Muscles with predominantly stability role characteristics (one-joint) optimally assist postural holding/anti-gravity/stability and control function. Muscles that have a stability function (one-joint stabiliser) demonstrate a tendency to inhibition, excessive flexibility, laxity and weakness in the presence of dysfunction (Kendall et al 2005). Janda (1983) described these muscles as ‘phasic’ muscles. 2. Muscles with predominantly mobility role characteristics (multi-joint) optimally assist rapid/accelerated movement and produce high force or power. Muscles that have a mobility function (two-joint or multi-joint mobiliser) demonstrate a tendency to overactivity, loss of extensibility and excessive stiffness in the presence of dysfunction (Kendall et al 2005). Janda (1983) described these muscles as ‘postural’ muscles. Bergmark (1989) developed a model to describe the muscle control of load transfer across the lumbar spine. He introduced the concept of local and global systems of muscle control. The local and global muscle system characteristics and general features are described in Table 2.2 with examples. 3. Local muscle ‘system’: the small deep segmental muscles in the local muscle system are responsible for increasing the segmental stiffness across a joint and decreasing excessive intersegmental motion. The relevance of this is that these muscles are ideally situated to control displacement of the path of the instantaneous centre of motion and reduce excessive intersegmental translatatory motion during functional movements. At end range of motion the passive restraints of motion (e.g. ligaments and joint capsules) contribute significantly to controlling translatatory or accessory motion. Local muscles maintain this translatatory control during all functional activities such as postural control tasks, non-fatiguing functional movements, fatiguing high load and high speed activities. Local muscles maintain activity in the background of all functional movements. Their recruitment is independent of the direction of loading or movement and is biased for non-fatiguing low load function, although they maintain the role of controlling intersegmental displacement during fatiguing high load function as well. The local muscles do not significantly change length during normal activation and therefore do not primarily contribute to range of motion. The one-joint (monoarticular) global muscles have a primary stability role, while the multi-joint (biarticular) global muscles have a primary mobility role. 4. Global muscle ‘system’: the muscles that make up the global muscle system are responsible for the production and control of the range and the direction of movement. The global muscles can change length significantly and therefore are the muscles of range of motion. The global muscles participate in both non-fatiguing low load and fatiguing high load activities. The functional efficiency of a muscle is related to its ability to generate tension. A muscle’s tension is not constant throughout a contraction, especially if the muscle is changing length to produce movement. Length and tension properties of a muscle are closely related. The tension or force a muscle produces is the resultant force arising from a combination of both active and passive components of the muscle. The active component of muscle tension is determined by the number of actin–myosin cross-bridges that are linked at any point in time. The passive tension property of muscle is largely due to the elastic titin filaments which anchor the myosin chain to the Z band. Other connective tissue structures within muscle only contribute partially to passive tension. Figure 2.1 illustrates the actin–myosin filament cross-bridges and titin attachments. The position in range (usually mid-range) where the active length–tension curve is maximal is known as the muscle’s resting length. In this position, the maximum number of actin–myosin cross-bridge links can be established. In a muscle’s shortened or inner range position, the passive elastic components do not contribute to muscle tension. Passive tension only begins to play a role after a muscle starts to lengthen or stretch into the muscle’s outer range, beyond its resting length or mid-range position. Muscles are most efficient and generate optimal force when they function in a mid-range position near resting length. Muscles are less efficient and appear functionally weak when they are required to contract in a shortened or lengthened range relative to their resting length because of physiological or mechanical insufficiency (Figure 2.2). However, when a muscle habitually functions at an altered length (either lengthened or shortened), its length–tension relationships adapt accordingly. The position in range where it generates optimal force efficiency changes to match the subsequent lengthening or shortening (Goldspink & Williams 1992), as illustrated in Figure 2.2. When a muscle is persistently elongated or lengthened, it adds sarcomeres in series (the broken line in Figure 2.2). Because the sarcomeres are the force generating units within a muscle, a lengthened or elongated muscle is stronger and is able to generate a higher peak force than normal. This higher peak force, however, is produced in an outer range position and not at its usual resting length, mid-range position. At the muscle test position (inner to middle range), the lengthened muscle is inefficient due to physiological insufficiency, and consequently tests ‘weak’ during muscle testing and fatigues more readily in postural control tasks. A persistently shortened muscle, on the other hand, loses sarcomeres in series and increases in connective tissue (the dotted line in Figure 2.2). Because of the reduced number of sarcomeres, the shortened muscle generates less peak force than normal. Interestingly, a shortened muscle’s resting length may coincide with the muscle test position. Even though the shortened muscle is weaker than its normal control, muscle testing is performed at the point in range where it is optimally efficient. Consequently, shortened muscles frequently demonstrate good strength during muscle testing (Gossman et al 1982). This explains the clinical observation that ‘short muscles test strong and long muscles test weak’. The concepts of local and global muscle systems and stabiliser and mobiliser muscles provide useful frameworks to classify muscle function. However, alone, they have some clinical deficiencies. By interlinking these two concepts a clinically useful model of classification of muscle functional roles has been developed (Comerford & Mottram 2001). Table 2.3 summarises this classification in terms of function and characteristics and dysfunction. Table 2.3 Classification of muscle functional roles in terms of function, characteristics and dysfunction Postural adjustments are anticipatory and ongoing and all muscles can have an anticipatory timing to address displacement and perturbations to equilibrium. All muscles provide reflex feedback reactions under both low and high threshold recruitment tasks and demonstrate anticipatory feedforward recruitment when appropriate. However, only muscles with a local stability role exhibit anticipatory timing that is independent of the direction of loading or displacement. Muscles recruited in a global range related role are direction-specific in their anticipatory feedforward responses (Hodges & Richardson 1997; Hodges 2001; Hodges & Moseley 2003). Figure 2.3 illustrates an example of the anatomical inter-relationships between the different muscle roles in the lumbar spine. Although all muscles can perform all basic abilities, some muscles are ideally suited to some roles to achieve optimal function better than others. An analysis of a muscle’s ideal role should consider the co-relation of the features listed in Table 2.4. This model of reviewing and analysing muscle function and recruitment provides an opportunity to develop a greater understanding of a muscle’s role in functional activities. By analysing the inter-relationships between a muscle’s anatomy and histology, its biomechanical potential, its recruitment physiology and consistent changes in the muscle related to pain and pathology (see Table 2.4), we can be more critical of some of the oversimplified roles that have previously been ascribed to some muscles. 1. Some discrepancies between biomechanics and neurophysiology need to be explained with some muscles. For example, training latissimus dorsi co-activation with the contralateral gluteal muscles (often referred to as the posterior sacroiliac sling) to stabilise the sacroiliac joint has been proposed by various authors (Vleeming et al 2007). This training would be appropriate to help manage sacroiliac joint pain associated with high load or high speed activities such as running or throwing because these two muscles are automatically recruited in these activities. However, for patients who have sacroiliac joint pain associated with non-fatiguing functional movements (e.g. normal gait) and postural control activities (e.g. static standing), this training is unlikely to be beneficial. There is often an assumption that the muscles used in strength training will be used in all functional activities. However, this is not the case as there is minimal automatic activation of latissimus dorsi in these low load activities. Another example of measurement discrepancy occurs following the assumption that psoas major is a hip flexor. Biomechanical modelling of psoas major often assumes that it is a fusiform muscle with a straight line of action from the upper lumbar spine to the femur. This is not the case. Psoas major is a pennate muscle with obliquely orientated fibres. A more detailed mechanical evaluation of its pennate orientation (Gibbons 2007) suggests that its maximum shortening potential is approximately 2.25 cm. This is insufficient to produce the flexion range of motion of the hip. The posterior fascia of psoas major is anchored to the anterior rim of the pelvis (Gibbons 2007). This attachment would produce posterior tilt of the pelvis. Posterior tilt of the pelvis is a conjoined movement with hip flexion, and interestingly the range of movement of the pelvis at the psoas attachment point during posterior tilt perfectly matches the predicted range of shortening of psoas major. 2. There may be misinterpretation of research measurement technology; for example, upper trapezius, lower trapezius, psoas major, vastus medialis obliquus. Upper trapezius has been assumed to elevate the shoulder because it demonstrates high levels of EMG activity during scapular elevation tasks. Johnson et al (1994) demonstrate that 90% of the contractile fibres of upper trapezius insert on the ligamentum nuchae below C6 and are horizontally orientated (the vertical fibres are predominantly fascial and connective tissue). They state that the upper trapezius muscle cannot elevate the scapula above C6. It is suggested that the reason for the high levels of EMG activation may be to assist the clavicular rotation (necessary for full shoulder elevation), or an attempt to stabilise the cervical spine during arm load activities. Similarly, vastus medialis obliquus demonstrates high levels of EMG activity in terminal extension of the knee. Lieb & Perry (1968) demonstrated that vastus medialis obliquus has no biomechanical potential to extend the knee in this last 30°. The high level of vastus medialis obliquus recruitment is best explained by its role of maintaining alignment tracking of the patella during the last 30° of full extension. 3. The muscle is designed to participate in more than one primary functional role; for example, Hodges (2003) suggests that a muscle may have three functional roles: Some muscles can effectively perform all three of these functional roles. Gluteus maximus is an example of a muscle that multitasks all three functional roles (Gibbons 2007). Gluteus maximus has deep sacral fibres that run from the inferior lateral corner of the sacrum to the posterior inferior ischial spine. It is believed that these fibres perform a local stability role and have the function of controlling intersegmental translation at the sacroiliac joint. Gluteus maximus also has fibres that run from the medial aspect of the ileum to the gluteal trochanter on the femoral neck. These deep fibres constitute the one-joint part of a muscle that performs a global stability role at the hip joint. The most superficial fibres of gluteus maximus run from the iliac crest and attach into the posterior aspect of the iliotibial band and eventually insert on the anterior aspect of the lateral tibial condyle, below the knee. This multi-joint part of gluteus maximus has a global mobiliser role and produces movement at the hip joint and the knee joint.
Muscle function and physiology
Introduction: Movement Control
Analysis of muscle function
Stabiliser and mobiliser function
Implications of stabiliser–mobiliser characteristics
Local and global function
Implications of local and global characteristics
Functional efficiency
Functional classification of muscle roles
Muscle characterisation
Stay updated, free articles. Join our Telegram channel
Full access? Get Clinical Tree
Muscle function and physiology
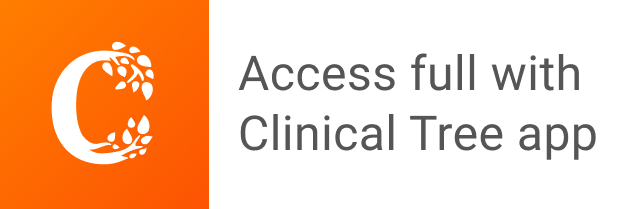