Neuromuscular diseases (NMD) constitute a group of phenotypically and genetically heterogeneous disorders, characterized by (progressive) weakness and atrophy of proximal and/or distal muscles. The objective of molecular testing is to confirm the pathogenicity of a relevant sequence variation by correlating an individual’s phenotype with what is expected in a given condition. Within the last two decades the application of molecular genetic strategies has led to a delineation of subgroups of clinically indistinguishable NMDs and has disclosed marked disease overlap. The expanding number of molecular defined NMDs requires new strategies to classify overlapping and clinical indistinguishable phenotypes.
Rapid and accurate diagnosis of diseases is very important for the appropriate treatment of patients. Recent advances at the molecular level and detection technologies are upgrading clinical diagnostics by providing new ways of diagnosis, with higher speed and accuracy. The recently developed molecular diagnostic assays based on the amplification, restriction maps, or hybridization of probe nucleic acids with target nucleic acids from clinical samples are allowing effective detection of various diseases with higher sensitivity and specificity compared with the other techniques used to date. Several applications of DNA microarrays for diagnosing specific diseases have been reported: genotyping and determination of disease-relevant genes or disease-causative agents, mutation analysis using relatively low-density DNA microarrays, single nucleotide polymorphisms (SNPs) screening using high-density DNA microarrays, analysis of copy number changes at the level of chromosome using comparative genomic hybridization DNA microarrays (arrayCGH), and global determination of posttranslational modifications including methylation, acetylation, and alternative splicing. Molecular genetic advances are creating a dynamic state of ever-expanding disease classifications for inherited and metabolic neuromuscular disease. Early neuromuscular disease classification systems were predicated on clinical features and patterns of inheritance. However, the nomenclature used to categorize various disease phenotypes is inconsistent, because some may be subclassified according to age of disease onset whereas others may be subclassified according to electrodiagnostic features.
Neuromuscular diseases (NMD) constitute a group of phenotypically and genetically heterogeneous disorders, characterized by (progressive) weakness and atrophy of proximal and/or distal muscles. The objective of molecular testing is to confirm the pathogenicity of a relevant sequence variation by correlating an individual’s phenotype with what is expected in a given condition. If a novel nonsynonymous mutation is identified then possible pathogenicity is determined by genotype-phenotype correlations within the family, if this is possible. Software programs such as Polyphen and SIFT offer first-pass assessments of whether the mutation would be tolerated by the protein, but these are only proxy estimates of pathogenicity. However, clinical diagnosis based on physical examination, family history, and noninvasive procedures is very important for the comprehensive evaluation of patients with NMDs, and molecular testing does not replace it.
Molecular diagnosis of NMDs is based on the nature of the sequence variation and the specifics of the genomic changes under consideration that dictate the method used for the investigation. For example, large variations, such as duplication of a segment of a chromosome as present in Charcot-Marie-Tooth disease type IA, are visible with appropriate labeling methods under a microscope, whereas small variations, such as a single base pair substitutions or mutations, might require sequencing for detection. For autosomal-recessive and X-linked disorders, diagnostic testing methods can be different for symptomatic individuals than for carriers, if the method only determines the presence, not the quantity, of mutant alleles ( Table 1 ).
Method | Description | Advantages/Disadvantages |
---|---|---|
Chromosome | ||
Fluorescent in situ hybridization (FISH) | Visualization of normal/abnormal DNA sequences | Detects mosaicism |
DNA a | ||
Restriction fragments (RFLP) | DNA fragments separated by electrophoresis | Requires mutation in restriction site to be known |
PCR used with other techniques | Creates multiple DNA copies | Does not detect different mutations from each other |
Sequencing | Gets DNA detail sequence | Does not detect deletions/duplications |
Denaturing techniques (DGGE, SSCP) | Short DNA segments separated by mobility | Does not distinguish between polymorphism and mutation |
Protein truncation test | In vitro gene expression | Detects non-sense mutations |
mRNA | ||
Northern blot | Separates mRNA | Determines cell type DNA expression |
Microarray expression analysis | Quantifies mRNA copies | Determines level of gene expression |
Protein | ||
Immunohistochemistry | Antibodies against target protein | All of them might be biased because enzymatic defect can be the consequence of other protein defect |
Enzyme functional analysis | Protein activity | |
Western blot | Separates proteins by mobility |
a DNA usually is genomic but mtDNA can be used. These test were explained in the first section of this article.
Modes of inheritance
Three different modalities of genetic transmission have been described for nuclear DNA. Autosomal recessive, in which the two different forms of the same gene (ie, alleles), each inherited from a different parent, are affected by a pathogenic mutation to cause the disease. For instance, an autosomal-recessive limb girdle muscular dystrophy (LGMD) in members with the same mutation present in the homozygous state (two copies). Autosomal dominant is the result of a mutation in a single gene or allele. Passing on the mutant allele transmits the disease to the offspring. A good example is autosomal-dominant tibial muscular dystrophy in members with heterozygous mutations (one copy), affecting the same gene that produces LGMD. X-linked disorders are associated with mutation in the X-chromosome and might be compromising 1 or 2 alleles depending of the severity of the mutation. For example, some X-linked dystrophin and probably most GJB1 mutations (causing X-linked Charcot-Marie-Tooth disease) are symptomatic in females. These are very simple rules; however, to complicate genetic disorders it is becoming more clear that a single mutation can be associated with different phenotypes depending on other regulatory phenomena such as epigenetics; that is, hypomethylation may account for some patients with facioscapulohumeral muscular dystrophy (FSHD) who have no abnormalities in their genomic sequences and environmental factors. Similarly, interaction between different mutated genes can have substantial consequences, in contrast to when only one of them is affected. For example, some mutations in the 3 collagen VI subunit genes (COL6A1, COL6A2, and COL6A3) produce autosomal-dominant Bethlem myopathy whereas other mutations in different locations in these genes produce autosomal-recessive Ullrich congenital muscular dystrophy.
Mitochondrial inheritance is the asexual transmission of mtDNA from a mother to her children. As all children of a woman inherit the same mtDNA sequence, there is generally in maternal inheritance a high recurrence risk in the future siblings of an affected child, often approaching 100%, although a high degree of clinical variability is common. Cells contain hundreds or thousands of mitochondria (skeletal muscle studies found a mean of 3650 mitochondrial genomes per 2 nuclear genomes) and each mitochondrion has multiple (estimates of 2–10) mtDNA genomes. mtDNA point mutations that are present in blood do not always reach the threshold for clinical disease in some or all of the matrilineal relatives, who can be asymptomatic or oligosymptomatic. Also, there are cases of nuclear DNA mutations affecting the protein activity of enzymes that play an important role in mitochondrial function. Around 100 nuclear genes have been described with mitochondrial functions, such as governing mitochondrial structure, function, replication, and protein import. Therefore, mitochondrial disorders can also be autosomal dominant or recessive, for example, autosomal dominant progressive external ophthalmoplegia or autosomal recessive progressive external ophthalmoplegia.
Laboratory investigations
The diagnosis of inherited and metabolic disorders is challenging enough, and it is very difficult to confirm them based on clinical findings or routine chemistry analyses. Usually the evaluation of individuals at risk includes investigations such as biochemical, electrodiagnostic, histopathologic, and molecular studies. The purpose of this review is to focus on molecular diagnosis.
Molecular testing is useful in specific disorders in individuals with preliminary testing, suggesting the possibility of well-characterized disorders including conditions associated with point mutations, such as mitochondrial disorders like NARP (neuropathy, ataxia, and retinitis pigmentosa), MERRF (myoclonic epilepsy with ragged-red fibers), or MELAS (mitochondrial encephalopathy with lactic acidosis and stroke).
Laboratory investigations
The diagnosis of inherited and metabolic disorders is challenging enough, and it is very difficult to confirm them based on clinical findings or routine chemistry analyses. Usually the evaluation of individuals at risk includes investigations such as biochemical, electrodiagnostic, histopathologic, and molecular studies. The purpose of this review is to focus on molecular diagnosis.
Molecular testing is useful in specific disorders in individuals with preliminary testing, suggesting the possibility of well-characterized disorders including conditions associated with point mutations, such as mitochondrial disorders like NARP (neuropathy, ataxia, and retinitis pigmentosa), MERRF (myoclonic epilepsy with ragged-red fibers), or MELAS (mitochondrial encephalopathy with lactic acidosis and stroke).
DNA and RNA investigations
Molecular testing at this level for inherited disorders can be divided in 4 categories: prenatal, carrier, susceptibility testing, and diagnostic. Usually ordered to confirm a diagnosis and requiring in general just a blood sample, molecular investigations do not always reach an accuracy of 100%. In fact, disorders with single mutations that are not well characterized or associated with a particular disorder only explain 60% to 70% of the causative mutations; therefore, a negative result for these disorders does not completely rule out the diagnosis.
There are different methods used to detect known and novel mutations. To determine which particular method is better, it is important to determine the potential size of the defect, whether DNA or mRNA should be used, and the type of tissue to analyze. The physical principles explaining the differences between mutant and wild-type DNA are based on (1) differences in electrophoretic mobility, (2) the ability of restriction enzymes from bacteria to recognize specific sequences and cleavage the double-stranded DNA, and (3) inhibition of formation of DNA duplex hybrids with synthetic probes.
Detection of known mutants
Polymerase Chain Reaction
The 1993 Nobel Prize in Chemistry was awarded to Dr Kary B. Mullis for the invention of the polymerase chain reaction (PCR), a method that made it possible to copy a large number of DNA fragments in only a few hours. PCR can amplify very specific segments of the genome from 1 or 2 copies to a workable quantity of 1 million or more copies. An important limitation of PCR is that the size of a DNA segment that can be amplified, which is limited to approximately 3000 base pairs, typically significantly fewer. A classic example is the amplification of genes associated with FSHD and spinal muscular atrophy (SMA), 2 genes that are similar but differ in the surrounding sequences, therefore making it impossible to amplify a big enough fragment to differentiate between them. Similarly, it does not distinguish among the presence of 1, 2, or 3 copies, typically related with duplication of genes ( Fig. 1 ).
Restriction Fragment Length Polymorphism
Restriction fragment length polymorphism (RFLP) is a procedure based on the recognition of amplified genomic DNA of the allelic variation of DNA or mutant genotype by bacterial enzymes that recognize specific sequences of DNA, cutting them, and producing DNA fragments with different sizes, which can be further separated by electrophoresis. The recognition is based on uncleaved DNA fragment that was not recognized by the bacterial enzyme, giving the change in sequence. Therefore, the mutant DNA will (ie, mutation causes new restriction site) or will not (ie, mutation causes loss of a restriction site) be cut by the restriction endonuclease. This differential pattern of cutting or generation of fragments of different lengths, when compared with the wild-type or normal DNA fragments, explains how this technique was named ( Fig. 2 ).
Molecular Cytogenetics
These studies provided early “physical maps” of chromosomes, and allowed for the direct visual identification of many genetic diseases in which sequence variations were of sufficient size to be recognized by abnormalities in the number, size, or pattern of bands present. Examples include various diseases with extra chromosomes (trisomies) or missing chromosomes. Molecular methods using chromosomal probes, complementary nucleic acid sequences binding directly to a short segment of a chromosome in defined location, produce higher resolution visualization. This technique, known as fluorescent in situ hybridization (FISH), uses fluorescent probes to detect sequence variations or mutants. This method has been applied to the diagnosis of CMT1A and hereditary neuropathy with liability to pressure palsy, disorders in which there is either a large duplication or deletion of a chromosomal segment, and for dystrophin analysis ( Fig. 3 ).
Allele-Specific Oligonucleotide Analysis
Allele-specific oligonucleotide analysis is based on amplification of DNA from the patient and hybridization with probes (oligonucleotides), each one of them testing for specific point mutations. It can evaluate up to 20 different point mutations in a target DNA. These probes, which are single-strand DNA, are immobilized in a solid support and then incubated with denaturated DNA from the patient, in an attempt to find a complementary sequence that binds covalently to the probe. This methodology has proven to be more cost-effective than RFLP analysis, especially in analyzing mtDNA, because it can detect 18 different mtDNA point mutations simultaneously ( Fig. 4 ).
Sequencing
The goal is to determine the sequence of a specific gene base by base. This procedure is based on PCR amplification of patient’s DNA, and the sample is run on a polyacrylamide electrophoresis gel able to separate fragments by size, even with just one base of difference, and determine the sequence of the gene. Sometimes sequencing may result in diagnostic uncertainties when the gene of interest has highly similar sequences elsewhere in the genome ( Fig. 5 ).
New mutation detection
Denaturing gradient gel electrophoresis and temperature gradient gel electrophoresis
These two similar techniques are based on the denaturing properties of DNA molecules. The extent of denaturation is dependent on its sequence, and it varies with temperature and chemical conditions. The amount of separation from double-stranded DNA to single-stranded DNA is inversely proportional to its mobility in gel electrophoresis. Whereas Denaturing gradient gel electrophoresis (DGGE) uses electrophoresis through a gradient of increasing chemical denaturant to separate different mutants of genes, temperature gradient gel electrophoresis (TGGE) uses a temperature gradient analogously. DGGE has been used for analysis of dystrophin mutations ( Fig. 6 ).
Indications for genetic testing in neuromuscular disease
Specific indications for genetic testing are not clear. The following comprises a guide to decision-making specific to patients with neuromuscular disease. Genetic testing is always helpful in establishing the diagnosis, and if the result is negative it is helpful to redirect which kind of investigations should be ordered in the future. Genetic testing may be helpful in the management of patients. Although there is no cure for any inherited neuromuscular disease, there are treatments in the fullest meaning of this term. For example, ensuring close cardiac follow-up and monitoring for heart block with timely insertion of pacemakers in patients with myotonic dystrophy can be life saving, and prevents further unnecessary testing such as muscle biopsy or imaging studies. More importantly, it avoids non-necessary treatments previously seen, such as esophageal procedures in patients with myotonic dystrophy and oculopharyngeal muscular dystrophy, and lumbar laminectomies and orthopedic leg procedures in patients with inherited neuropathies ( Fig. 7 ).
Applications
The current nomenclature system for inherited and metabolic myopathies is increasingly blurred by progress in their clinical and molecular understanding. For instance, the presence of “dystrophic” features within muscle biopsies, such as large hypertrophied myofibers and increased connective and fatty tissues, characterized dystrophies, whereas congenital myopathies were categorized as relatively nonprogressive early-onset disorders with specific, nondystrophic pathologic features. Furthermore, congenital muscular dystrophies were so termed because of the dystrophic features on muscle histochemical studies and their frequent presence in infancy. However, some dystrophies do not have dystrophic features on muscle biopsies (eg, some LGMDs, and myotonic dystrophy types 1 and 2), congenital myopathies may have adult onset (eg, nemaline myopathy) and progression, and distal myopathies may also have proximal weakness and be classified as LGMDs (dysferlin mutations).
Dystrophinopathies
The muscular dystrophies known as dystrophinopathies are classically characterized by 3 main clinical syndromes: Duchenne muscular dystrophy (DMD), Becker muscular dystrophy (BMD), and X-linked dilated cardiomyopathy. Clinically some dystrophin mutations can produce syndromes that are mistaken for other dystrophies, including LGMD and facioscapulohumeral muscular dystrophy.
The dystrophin gene is one of the largest known human genes. It contains 79 exons and its total size is around 2.4 million base pairs, approximately one-thousandth of the entire genome sequence. Therefore, testing for mutations is particularly complex. Roughly 60% of patients with DMD have large deletions of one or more exons, 35% have small or point mutations, and 5% have large duplications or chromosomal rearrangements. Most large deletions are detected by RFLP analysis. Because most deletions tend to occur in the same exons, called hot spots, PCR amplification and sequencing of limited numbers of exons is a suitable efficient technique and is able to detect almost all large deletions. The most difficult task is detection of small mutations, which have to be detected by sequencing of multiple exons. Denaturing techniques (single-strand conformation polymorphism, DGGE) detect 90% to 100% of mutations, though they will also detect other sequence variations not considered mutants, and require further analyses, usually sequencing, to determine whether the variation is a benign polymorphism or mutation. Protein methods and muscle immunohistochemistry with antibodies directed against dystrophin may show absence of muscle dystrophin in DMD males and mosaic patterns in female carriers; however, genetic testing is more accurate.
Limb girdle muscular dystrophies
Clinically characterized by predominant involvement of proximal arm and leg muscles, with type I being autosomal dominant and type II autosomal recessive, usually the LGMD phenotypes are not distinctive enough to allow for the use of DNA diagnostic testing as a first step. However, particular mutations may present with distinctive features, such as with dysferlin (very high serum creatine kinase [CK]; prominent calf atrophy and weakness), caveolin-3 (coexisting rippling muscle disease), lamin A/C (early elbow flexion and ankle plantar flexion contractures, cardiac conduction defects), and calpain-3 (prominent scapular winging, weakness of shoulder adduction and elbow flexion, contractures, and severe posterior thigh involvement). At present, the molecular diagnosis of most LGMDs is based on protein analysis from muscle biopsies with immunohistochemistry or Western blot, sometimes followed up with DNA testing. However, immunohistochemistry results must be interpreted cautiously, as a genetically based reduction in one sarcolemmal protein may result in secondary reduction in several other proteins and therefore confound interpretation. For patients with a family history suggesting an autosomal-dominant limb girdle myopathy, lamin A/C mutations in blood specimens should be sought prior to muscle biopsy. By contrast, patients with a family history suggestive of autosomal-recessive inheritance might have sequential blood genetic testing for dysferlin (by Western blot), calpain-3, and fukutin-related protein, and only undergo muscle biopsy if these are negative ( Table 2 ).
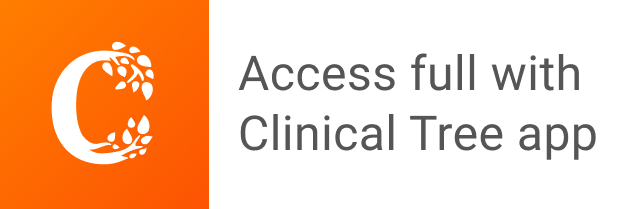