Fig. 6.1
Illustration of meniscus showing generalized anatomic macrostructure (a), wedge-like cross section displaying a simplified collagen fiber organization, with the majority of fiber bundles in the circumferential direction with occasional radial “tie” fibers (b), and forces acting on the meniscus producing both compressive and tensile loads (c) (F and T correspond to forces from the femur and tibia, respectively. PD and ML represent the proximal-distal and medial-lateral directions, respectively) (Adapted from Fisher et al. [72] with permission from Elsevier)
Many replacement scaffolds are designed to degrade overtime and be replaced by host tissue or, if containing a cellular component, to remodel substantially. The long-term success of these scaffolds will be dependent on their ability to induce a new, living tissue with structure similar to the meniscus, which is complex and performs many diverse functions. A brief description of the components of native meniscal tissue will help illustrate the challenges for proper scaffold creation. Meniscus fibrochondrocytes (MFCs) sparsely populate the native tissue and function to maintain and remodel the extracellular matrix (ECM) [20, 21]. Proteoglycans are concentrated in the inner regions where compressive loading predominates [20, 21]. This region also features a mixture of type I and II collagen [20, 21]. Within the outer region, type I collagen predominates to support tensile loading. These collagen fibers are mostly oriented in the circumferential direction (Fig. 6.1), with the occasional radial “tie” fiber or sheet interspersed within this network [22]. The architecture of this collagen component is paramount for meniscus mechanical function. When compressive loads impinge on the wedge-shaped meniscus, an outward tensile load is generated (Fig. 6.1). The circumferential collagen fibers resist these “hoop stresses” and enforce joint congruency and promote axial load transmission. The success of any “nonpermanent” scaffold will be dependent on its ability to promote new tissue formation with these structural features and hierarchical arrangements.
Types of Materials and Fabrication Techniques
For meniscus replacement scaffolds, two broad classes of materials have been used: synthetic and natural (see Table 6.1 for a list of commonly used materials and fabrication techniques). Synthetic materials consist mainly of polymers, which are biocompatible, easily manufactured, and feature appropriate and modifiable mechanical properties. These include polyvinyl alcohol (PVA), Dacron, polyurethane, polyglycolic acid (PGA), poly-l-lactic acid (PLLA), and polycaprolactone (PCL), among others [23–32].
Table 6.1
Common materials and fabrication techniques used for meniscus scaffolds
Base material | Fabrication techniques | |
---|---|---|
Synthetic | Poly(ε-caprolactone) | |
Polyvinyl alcohol | ||
Polyurethane | ||
Polyglycolic acid | ||
Poly-l-lactic acid | ||
Natural | Collagen (type I, type II, types I/III) | |
Hyaluronan/hyaluronic acid | ||
Fibrin | Sealant/hydrogel [74] | |
Chondroitin sulfate | ||
Agarose | Hydrogels [46] | |
Alginate | ||
Cellulose | Hydrogels [43] | |
Chitosan | ||
Silk | Porous foams [45] | |
Small intestinal submucosa | ||
Perichondral matrix | ECM as is (decellularized) [34] |
Alternatively, investigators have created scaffolds made from natural components of the extracellular matrix (ECM). These include tissues directly derived from donors, such as porcine small intestinal submucosa (SIS) [33] or perichondral tissue from the rib [34]. These substances hold the promise of facile incorporation and assimilation. For instance, SIS has shown superiority in cellular interactions versus dermal scaffolds [35]. These submucosal tissues are obtained, decellularized, lyophilized, sterilized, and packaged for direct implantation. One issue is that the decellularization process could result in loss of important biochemical components, such as glycosaminoglycans (GAGs), and as a result, reduced biomechanical properties. As such, a number of “kinder” decellularization techniques are being evaluated [36, 37]. In addition, natural ECM components can be isolated, purified, and utilized as base components for use in similar fabrication methods as synthetic materials. These include type I collagen, glycosaminoglycans, fibrin, and hyaluronic acid [12, 38–41]. As these ECM components naturally interact with cells, the biocompatibility and bioactivity of scaffolds made from such molecules is superior. However, the full complexity of the native ECM environment is likely not achieved. Alternative biological polymers commonly used are agarose, alginate, cellulose, chitosan, and silk [42–46]. Another method is to allow dense cell pellets to make their own matrix, generating a neo-tissue de novo from accumulated matrix molecules [23].
Using these base materials, a number of techniques can be used to form three-dimensional scaffolds. Foams can be formed by dissolving a polymer into a solution and then placing the solution into a mold and drying it, leaving the remaining polymer. By including a salt solution, pores can be created by placing the scaffolds in an aqueous solution and allowing the salt to be leached from the scaffolds. Alternatively, polymer fibers can be combined together into woven or nonwoven felts with micron-sized fibers using technology borrowed from the textile industry. For scaffolds with fibers on the order of nanometers, a process called electrospinning can be used. Finally, hydrogels are commonly made from a number of materials and feature a large water content, usually >90 %. These gels can be formed into any shape before gelling via changing temperature, pH, electric field, ultrasound, salt concentration, or ultraviolet light. Overall, with the right materials and fabrication methods, compressive properties can be obtained similar to the native tissue [23, 38]. Tensile properties are less widely reported; however, with the proper materials and fabrication methods, mechanical properties approaching that of the native meniscus are possible [47, 48]. Each method has advantages and disadvantages, which have been nicely summarized elsewhere [12, 49]. This chapter will focus on the implementation of the above described materials and fabrication methods to create and evaluate scaffolds for meniscal replacement.
Acellular Permanent Replacements
Early on, several acellular scaffolding materials were evaluated in initial preclinical animal studies, including PLLA foams embedded with carbon fibers [32], Dacron [30], and porous polyurethane [27]. The first alternative meniscal replacement to be used widely is the collagen meniscus implant (CMI), otherwise known as Menaflex® (ReGen Biologics, Inc.). This device is intended as an alternative for partial meniscectomy, especially with the presence of an intact peripheral rim. It was the first device to be approved by the Food & Drug Administration in the USA (although its approval has been rescinded due to an investigation of the initial approval [50]). This scaffold is produced from a slurry of decellularized bovine Achilles tendon and GAGs, which is molded into a semilunar shape, then lyophilized, chemically cross-linked, and sterilized (Fig. 6.2) [40]. Preclinical data in the dog model suggest the scaffold can allow cellular ingrowth and induce new tissue formation in 63 % of animals at 1 year postoperatively (versus 25 % of no scaffold controls) [40]. Several clinical studies have been published using the CMI device [51–54]. One year after implantation, second-look arthroscopy revealed significant remodeling of the CMI [52]. Through biopsies, clear cellular infiltration and production of a fibrocartilaginous matrix at the edge of the scaffold has been observed. Yet, it is unclear whether this remodeling process occurs throughout the entire CMI.
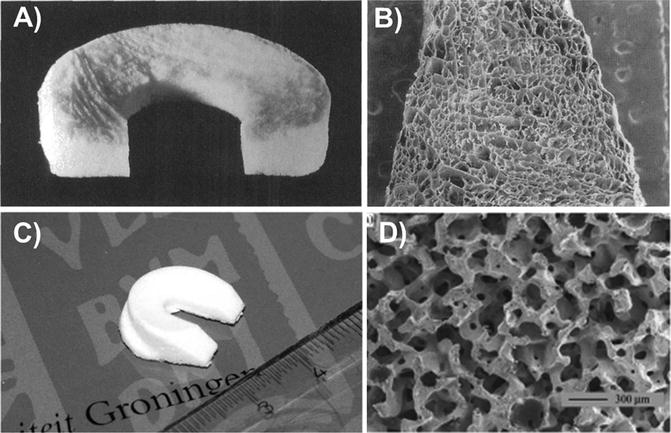
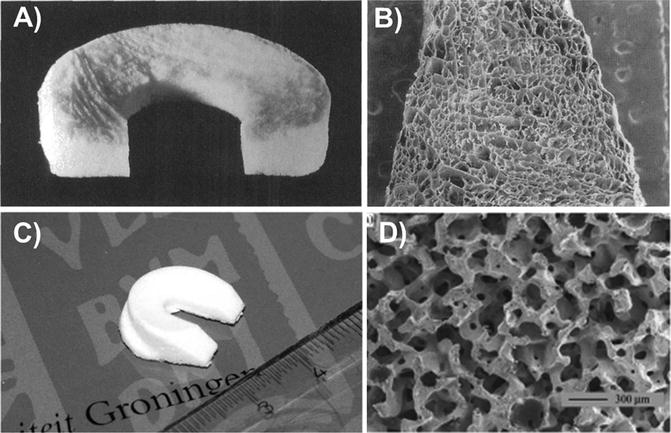
Fig. 6.2
Acellular scaffolds used clinically for meniscus replacement. Collagen meniscus implant (CMI) (a, b) and Actifit (c, d) scaffolds showing gross appearance (a, c) and microstructure (b, d) (Adapted from Stone et al. [92] with permission from JBJS and Heijkants et al. [25] with permission from Springer)
The most well-known randomized control trial evaluating CMI was performed by Rodkey et al. [52–54]. In over 300 patients, the CMI was directly compared to treatment with a partial medial meniscectomy. Second-look arthroscopy at 1 year postoperatively showed that treatment with the CMI led to significantly more new meniscal tissue compared to partial meniscectomy, and the implant itself was integrated. Interestingly, patients with chronic tears had significantly higher activity levels relative to controls and had lower rates of revision surgeries at an average of 5 years follow-up. However, patients with acute injuries showed no statistically significant impact of the CMI. In addition, there were no reports of chondral wear due to the implant. However, this study has been criticized for certain methodologic flaws. Recently, Monllau et al. have reported long-term outcomes (>10 years) following CMI treatment in a series of 25 patients [51]. Although not controlled, the study showed good or excellent results in 83 % of patients with improved activity and pain levels. However, magnetic resonance imaging revealed shrinking of the implant in 89 % of cases and a failure rate of 8 %.
A more recently developed synthetic scaffold (Actifit; Orteq Bioengineering) is currently on the market in Europe as an alternative for partial meniscectomy. This scaffold, based on a polyurethane/PCL mixture, is lyophilized into a foam-like open pore structure using liquid phase separation and salt leaching techniques, and the final product features a C-shaped geometry and cross-sectional wedge shape (Fig. 6.2) [25, 31]. In a skeletally mature sheep model [55], MRI and histological assessment revealed little to no cartilage damage under the site of scaffold implantation up to 1 year postoperatively. This is supported by in vitro studies in which a similar PCL scaffold could restore contact pressures under the meniscus in the sheep knee joint during a simulated gait movement [14]. Interestingly, however, cartilage damage was found near the midline of the joint in both the Actifit and partial meniscectomy groups, suggesting some altered loading patterns in vivo. These results are mirrored in the dog model of complete meniscus replacement, with cellular infiltration and matrix formation observed at 12 weeks following implantation [25], but a substantial amount of articular cartilage damage found by 6 months [56]. Compressive testing revealed that the behavior of the excised tissue better resembled the native tissue compared to the original scaffold, although additional improvements are needed to fully replicate the mechanical behavior of the natural structure. Moreover, longer term studies (2 years) showed that the core of the scaffold was acellular, although an ECM remained, bringing the long-term viability of the regenerated tissue into question [57].
The Actifit device was recently evaluated in a case series of more than 50 patients with a previous meniscectomy who still suffered from pain [58]. At 2 years postoperatively, clinical outcome scores (indicating pain, activity levels, and quality of life) were significantly improved relative to preoperative scores. However, failure was noted in 17 % of patients who required reoperation. Biopsy of the scaffold revealed tissue ingrowth and matrix production near the edge of the scaffold [59]. Recent clinical data also highlights the importance of an intact and thick peripheral rim for preventing radial displacement of these scaffolds, particularly when used for replacement of the medial meniscus [60]. With continued use, a controlled study comparing the implant versus meniscectomy is still needed to prove the efficacy of the Actifit scaffold.
Hydrogels made from a PVA hydrogel have also been well studied for meniscus replacement. PVA gels naturally swell in water, leading to high water content (>90 %) and compressive properties similar to the native meniscus. In a rabbit model, implantation of the PVA hydrogel could lessen the degree of cartilage damage up to 2 years compared to meniscectomy, although both groups showed degenerative changes [28]. The PVA hydrogel was able to maintain its mechanical characteristics; however, the ability of this scaffold to integrate with the surrounding tissue was questioned. Kelly et al. used a PVA hydrogel threaded with sutures in a circumferential manner in an ovine model to completely replace the lateral meniscus [61]. Relative to complete meniscectomy, use of the hydrogel led to less cartilage degeneration as assessed via MRI, biomechanical testing, and histology. However, at 4 and 12 months, the hydrogel replacement showed greater cartilage degeneration when compared to a meniscal allograft. In fact, by 12 months, all hydrogels had failed mechanically, with a complete radial fracture in the posterior third of the implant. This study highlights both the need for long-term follow-up in animal models (1 year or more) as well as scaffolds matching both the compressive and tensile properties of the native meniscus. As an initial step toward this goal, Holloway et al. have recently created PVA hydrogels reinforced with ultrahigh molecular weight polyethylene (UHMWPE) fibers to strengthen the tensile mechanics [48]. By modifying the percent composition of the PVA and UHMWPE as well as varying the number of freeze-thaw cycles during fabrication, the scaffolds possessed both compressive and tensile modulus similar to the native meniscus. However, these properties are likely isotropic, i.e., the same in all directions, unlike the native meniscus, which features vastly different properties in the radial and circumferential directions. Similarly, polycarbonate urethane (PCU) scaffolds reinforced with circumferential Kevlar© (DuPont) fibers have also been used in preliminary studies to replace the entire meniscus in adult sheep (n = 3) [62]. Still, long-term large animal studies with a meniscectomy as a control are needed to confirm the effectiveness of these scaffolds.
As a consequence of implant failures, significant efforts have been made in recent years to better match the tensile properties of the meniscus in replacement scaffolds. For example, Balint et al. developed a scaffold comprised of a type I collagen sponge reinforced with resorbable, yet stiff synthetic polymer fibers (poly(desaminotyrosyl-tyrosine dodecyl ester dodecanoate)) [63]. Biomechanical testing in an ex vivo joint model showed that the fiber-reinforced scaffolds could bear compressive loads via the formation of circumferential tensile loads with properties similar to those of the normal ovine meniscus. One limitation of combining materials of varying mechanical characteristics is the possibility of the strong fibers to pull through the potentially fragile hydrogel or foam phase. Another concern is that these large fibers do not mimic the size or number of the collagen fibers in the native meniscus.
One way to instill anisotropic tensile properties uniformly within scaffolds while mimicking the nanofibrous nature of the native ECM is through a process known as electrospinning [64–69]. In particular, electrospun scaffolds made from PCL can match the tensile properties of the native meniscus within the physiological levels of strain [70, 71], and the tensile properties in the aligned direction are more than an order of magnitude higher than in the perpendicular direction. To create scaffolds featuring fibers that are locally aligned but show a continuous change in macroscopic directionality, we recently developed a novel electrospinning method (using a rotating disc mandrel) to produce scaffolds composed of circumferentially aligned (CircAl) nanofibers (Fig. 6.3) [72]. These novel scaffolds, with spatially varying local orientation and mechanics, may enable the formation of functional anatomic meniscus constructs with locally varying fiber architecture to recapitulate native tissue properties.
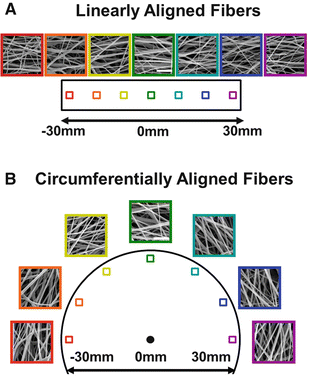
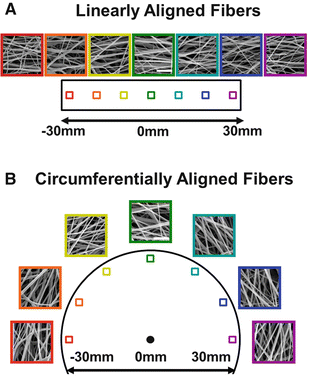
Fig. 6.3
Scanning electron microscopy for linearly aligned (a) and circumferentially aligned (b) scaffolds (scale bar = 5 μm) (Adapted from Fisher et al. [72] with permission from Elsevier)
Scaffolds for Healing and Regeneration
Instead of designing scaffolds for permanent replacement of the meniscus, some acellular scaffolding materials have been conceived with the purpose of stimulating a healing and regenerative response in vivo. By attracting cells through growth factors and stimulating them to produce new tissue, these scaffolds would be used as a temporary substrate, which would degrade and remodel as the new, and hopefully more permanent, adjunctive tissue forms.
One obvious scaffold is the fibrin clot, which is essential during normal tissue healing in the presence of a blood supply [73]. In pioneering work, Arnoczky et al. created a small full-thickness defect in the avascular portion of the meniscus in the dog model. Interestingly, the fibrin clot was able to support the formation of fibrocartilaginous tissue while untreated defects did not heal. The authors speculate that the reparative cells originated from the synovial membrane as well as the adjacent meniscal tissue. In later work, Hashimoto et al. added endothelial growth factor to a fibrin sealant and showed increased tissue filling [74]. Despite these positive findings, the histological appearance was still altered relative to normal. Additionally, the ability of a fibrin clot to heal large defects is unlikely.
Other biological scaffolds, such as SIS, have also been used as a graft to promote meniscal regeneration of meniscal defects. Five dogs received grafts and two were left untreated as controls (Fig. 6.4). In a small study in the canine model [75], implantation of the SIS encouraged substantially greater tissue formation at 12 weeks in 80 % dogs with a histological appearance similar to native tissue, including the presence of type II collagen, while untreated control defects filled with fibrous tissue. In a larger follow-up study at 12 months, SIS treatment led to reduced lameness and less secondary cartilage damage [33]. The increase in new tissue formation with SIS observed at 12 weeks was maintained (Fig. 6.4). This tissue was also more mature and better integrated. Still, changes to the articular cartilage were observed in both treatment groups. Additionally, the compressive stiffness of the regenerated tissue, even with SIS treatment, was half that of normal at 1 year follow-up.
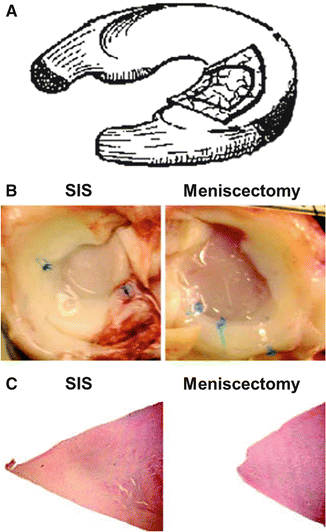
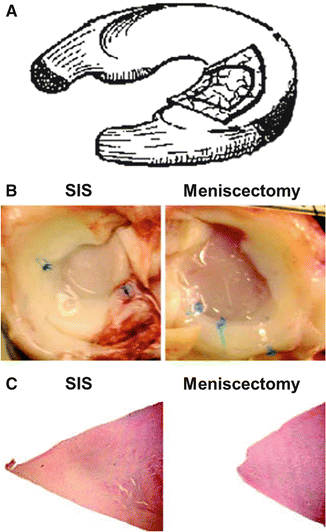
Fig. 6.4
Small intestinal submucosa (SIS) scaffolds for meniscus regeneration. Schematic of scaffold placement (a). Gross images (b) and histological staining (hematoxylin and eosin) (c) of SIS-treated and meniscectomy control menisci at 12 months (Adapted from Cook et al. [33] with permission from SAGE Publications)
Tissue-Engineered Scaffolds
Several research studies have suggested that acellular scaffolds (even ones promoting regeneration) are insufficient to allow incorporation, promote cell infiltration and new matrix production, and improve long-term function [76, 77]. As such, inclusion of cells within scaffolds and culturing in vitro to produce a tissue-like material prior to implantation has been widely pursued and is commonly known as tissue engineering. A number of cell sources are potentially available, including autologous meniscus cells, mesenchymal stem cells (MSCs), xenogeneic cells, embryonic stem cells, and induced pluripotent stem cells. The advantages and disadvantages of each cell type have been detailed elsewhere [12]. To date, no cell type has been approved for treatment of meniscal injuries, but much in vitro and preclinical in vivo work has been carried out to support their use.
In terms of in vivo evaluation, Ibarra et al. performed one of the earliest tissue engineering studies for the meniscus by seeding immature bovine fibrochondrocytes onto nonwoven PGA scaffolds followed by evaluation in the sheep model [26, 78]. More recently, the role of cells pre-seeded onto a scaffold was isolated and explored [76]. In an ovine partial meniscectomy model, the authors compared treatment with a porous hyaluronic acid/PCL scaffold (with PLA circumferential fibers) alone or seeded with autologous chondrocytes. At 1 year, cellular ingrowth was found from the capsule in all scaffold implants. Histological analysis found cells throughout all constructs (whether originally seeded or not), although a more rounded phenotype was observed in pre-seeded scaffolds (Fig. 6.5). Relative to meniscectomy, the cell-seeded group showed less osteoarthritic changes, although no statistically significant differences could be determined relative to the cell-free scaffold. Martinek et al. investigated the impact of pre-seeding the CMI device with autologous fibrochondrocytes for 3 weeks prior to autologous implantation in the sheep model [77]. After 3 months, the CMI decreased in size with or without cellular pre-seeding. The initial presence of cells also promoted ECM deposition. Interestingly, remodeling was observed in the majority of pre-seeded scaffolds with complete resorption in 25 % of animals. More conclusive in vivo studies are still needed.