Materials and Technology
Géza F. Kogler
Learning objectives
On completion of this chapter, the reader will be able to:
1. Compare and contrast the materials most often used in current orthoses and prostheses.
5. Delineate the steps in the fabrication or production of a custom orthosis or prosthesis.
A fundamental concept and common goal within the professions of orthotics, prosthetics, and rehabilitation is the restoration of normal form and function after injury or disease. To accept this challenge, the fields of orthotics and prosthetics have evolved into uniquely specialized professions. In addition to training in the basic biological and medical sciences, orthotists and prosthetists have an understanding of biomechanics, kinesiology, and the material sciences complemented by highly developed technical skills. Knowledge of the physical properties of materials and the techniques to manipulate and use them is essential to the design and fabrication of orthoses and prostheses. The topic is presented here as a general overview so that the rehabilitation clinician can develop a basic understanding of current design and fabrication processes used by orthotists and prosthetists.
Orthotics and prosthetics in the twentieth century
Orthotics and prosthetics have a rich history of research and development. Many innovative devices have been designed to restore function and provide relief from various medical ailments. Although progress can be documented throughout human history, the most significant contributions to orthotics and prosthetics were made in the twentieth century, stimulated by the aftermath of the world wars. Injured veterans who returned home from battle with musculoskeletal and neuromuscular impairments or traumatic amputation dramatically increased the demand for orthotic and prosthetic services. Although World War I stimulated some clinical progress in the two disciplines, notable scientific advancements did not occur until World War II. To improve the quality and performance of assistive devices at the end of World War II, particularly for veterans with amputation, the U.S. government sponsored a series of research and development projects under the auspices of the National Academy of Sciences (NAS) that would forever change the manner in which orthotics and prosthetics would be practiced.1
An extensive research effort was initiated by the NAS in late 1945, when a consensus conference revealed that few modern scientific principles or developments had been introduced in prosthetics.2 Research and educational committees were formed between 1945 and 1976 to advise and work with the research groups. Universities, the Veterans Administration, private industry, and other military research units were subcontracted to conduct various prosthetic research projects. In summarizing the most notable achievements in prosthetics during this period, Wilson3 cites the development of the total contact transfemoral socket; the quadrilateral socket design and hydraulic swing-phase knee-control units for the transfemoral prosthesis; the patellar tendon-bearing (PTB) transtibial prosthesis; the solid-ankle, cushioned-heel prosthetic foot; several new designs for the Syme’s prosthesis; and the Canadian hip-disarticulation prosthesis. He also notes the implementation of immediate postsurgical and early fitting as having a significant impact on the rehabilitation process for persons with lower extremity amputation. The most notable improvements in upper extremity prosthetics were the lyre-shaped three-jaw chuck terminal device and more efficient harnessing systems. In addition, modular components and advances in bioengineering have permitted increased use and availability of the myoelectric prosthesis since it was first proposed in 1950.4
Of the wealth of scientific advances made during this intensive research period, the most important is the greater attention paid to the biomechanics of prosthetic alignment and socket design.5 According to Wilson,2 “The introduction of socket designs based on sound biomechanical analyses to take full advantage of the functions and properties of the stump in conjunction with the rationale for alignment undoubtedly represents the greatest achievement in prosthetics since World War II.”
Although the focus of the NAS Artificial Limb Program was in prosthetics, it was anticipated that these efforts would also benefit orthotics. A formal research directive in orthotics did not begin until 1960. Biomechanical principles developed for the PTB prosthesis were immediately introduced in orthotics at the Veterans Administration Prosthetic Center, with the PTB orthosis to unload the foot-ankle complex axially.6 The concept of fracture bracing or cast bracing began at approximately the same time and is now common practice for orthopedic management of fractures.7,8 Clinical aspects of orthotic practice were also considered; a systematic approach to prescription formulation was established with the development of the technical analysis forms. Nomenclature to describe orthoses and their functions was standardized to identify the body segments they encompassed with the desired biomechanical control mechanisms.9
The introduction of new materials led to further advances in the field shortly after World War II. The use of thermosetting plastics in prosthetics permitted the development of the suction socket suspension system.10 Transparent plastics offered a new approach to diagnostic and fitting evaluation techniques, such as the transparent prosthetic socket (test socket) and the transparent face mask for patients with thermal injuries. In orthotics, the addition of thermoplastics led to numerous innovative designs of ankle-foot orthoses (AFOs) in the 1960s and 1970s. The custom plastic AFO was an important technological advance in lower extremity orthotics. The physical characteristics of thermoformable plastics allowed biomechanical controls to match the prescription for improved function. The mechanical properties of an orthosis could be controlled by the layout of the trimlines of a device or structural reinforcements through specially placed corrugations that could be incorporated into its surface geometry. Advances have been steady in the area of material engineering and continue to have an impact on orthotics and prosthetics. Numerous prosthetic feet have been introduced as elite athletes demand increased performance capabilities from their prosthetic components. Innovative designs for some prosthetic feet have been possible in part because of the diversity of carbon composite technology complemented by sound engineering design.
The development of computer-aided design/computer-aided manufacture (CAD/CAM) systems for orthotics and prosthetics, which began in the 1970s, was another major technological advance, considering the long tradition of custom hand-crafted devices in the profession. In the late 1980s and early 1990s, as computers became more economical, facilities began to integrate CAD/CAM systems into their practices. CAD/CAM systems have now been designed for most orthotic and prosthetic applications, often with specialized digitizers, scanners, and milling equipment to accommodate the unique needs of a particular device. The current trend within the profession is that orthotists and prosthetists use the CAD portion to digitize and manipulate the data, then subcontract the production of a device from a central fabrication company for the CAM portion. The art and workmanship that have distinguished the orthotists and prosthetists from other health professionals for most of the twentieth century continue to evolve as CAD/CAM technologies improve the design, manufacture, and diagnostic aspects of the field.
Orthotics and prosthetics have played an important historical role in the development of medical and surgical orthopedics and rehabilitation. Fundamental concepts that evolved from orthotic and prosthetic advancements are now basic principles in rehabilitation. Orthotics and prosthetics have evolved as sister professions because the technical skills and knowledge base to prescribe, fabricate, and fit the respective mechanical devices are similar. Because of this, material and technological advancements have been shared between these two rehabilitation specialties.
Materials
In the first part of the twentieth century, orthoses were constructed primarily of metal, leather, and fabric, and prostheses were manufactured from wood and leather. In the last 60 years, however, tremendous technological advancements have been made in the material sciences. The demand for strong and lightweight components in the aerospace and marine industries has produced a variety of new materials that possess mechanical properties suitable for use in the construction of orthoses and prostheses. New plastics have led to revolutionary advancements in the profession, permitting increased durability and strength and significant cosmetic improvements. Although a multitude of materials are now available, traditional ones are still in wide use; material selection depends in part on the individual needs of each patient. In a rehabilitation team setting, the orthotist and prosthetist are responsible for choosing the appropriate materials and components for fabrication because their experience and training are specialized in this area.
This chapter presents an overview of the general types of materials used in orthotics and prosthetics for rehabilitation professionals. Publications by the American Society for Testing and Materials contain specific technical information.11 Industry standards established by the International Organization for Standardization for consumer and patient protection give the strength requirements for orthotic and prosthetic components.12
The types of materials used most commonly in current orthotic and prosthetic practice include leather, metal, wood, thermoplastic and thermosetting materials, foamed plastics, and viscoelastic polymers. In deciding which materials are most appropriate for a patient, the orthotist or prosthetist considers the five important characteristics of materials: strength, stiffness, durability, density, and corrosion resistance.13
A material’s strength is determined by the maximum external load that the material can support or sustain. Strength is especially important in lower limb devices, in which loading forces associated with gait can be very high, or when heavy use of the orthotic or prosthetic device is anticipated.
Stiffness is the amount of bending or compression that occurs when a material is loaded (stress/strain or force/ displacement ratios). The stiffer a material, the less flexible it is and the less likely that deformation will occur during wear. When significant external stability is desirable (e.g., in a fracture brace or a rigid prosthetic frame), a stiff material is often chosen. When conformation to body segments is necessary (e.g., in a posterior leaf-spring AFO or a flexible transfemoral prosthetic socket), a more flexible material is used.
Durability (fatigue resistance) of a material is determined by its ability to withstand repeated cycles of loading or unloading during functional activities. Repeated loading compromises the material’s strength and increases risk of failure or fracture of the material. Fatigue resistance is especially problematic in the interface of materials with different characteristics.
Density is the material’s weight per unit of volume, a prime determinant of energy cost during functional activities while a patient wears a prosthetic or orthotic device. Although the goal is to provide as lightweight a device as possible, strength, durability, and fatigue resistance needs may necessitate a denser material.
Corrosion resistance is the degree to which the material is susceptible to chemical degradation. Many of the materials used for orthoses or prostheses retain heat, making perspiration a problem. For some patients who require lower extremity devices, incontinence may also be a concern. Materials that are impervious to moisture are easier to clean than porous materials.
The ease of fabrication is another important consideration for materials. Certain materials can be easily molded or adjusted for a custom fit; others require special equipment or techniques to shape the material.
Leather
Leather is manufactured from the skin and hides of various animals. Tanning methods and the type of hide determine the final characteristics of the leather. As an interface material for an orthosis or a prosthesis, vegetable-tanned leather is used to protect the skin from irritation. Chrome-tanned leather is used for supportive purposes when strength and resiliency are needed. Additional chemical processes can be incorporated during manufacturing to produce leathers that are waterproof, porous, flexible, or stiff. Useful qualities of leather include its dimensional stability, porosity, and water vapor permeability.14 These features have made leather a frequently used material within orthopedics, and it continues to be a material of choice in many current devices. Today, leather is used for supportive components such as suspension straps, belts, and limb cuffs. Leather is also used to cover metallic structures such as pelvic, thigh, and calf bands. For foot orthoses and shoe modifications, leather is often preferred over synthetic substitutes because of its superior “breathability” characteristics.
Another important attribute of leather is its moldability. Although numerous techniques are available to mold leather, the most common one in orthotics and prosthetics is to stretch it over a plaster cast after it has been mulled (dampened or soaked) in water. When the water evaporates from the molded leather, its dried shape is maintained, and the leather can be trimmed to the desired dimensions. To increase strength and durability, leather can be reinforced by lamination with plastics or other leathers. Similarly, if padding is desired over bony regions of the body, foamed plastics or felt can be sandwiched between layers of leather for comfort or to distribute applied forces over a larger surface area. Three basic skills are required for crafting orthotic or prosthetic components of leather: cutting, sewing, and molding. A technique specific to leather work is that of skiving, or thinning the edge on the flesh side of the hide. Finishing methods such as these contribute to the final appearance of the leather work and the device.
Metals
The types of metal used in the fabrication of orthoses and prostheses can be categorized into three groups: steel and its alloys, aluminum, and titanium or magnesium alloys. These metals may or may not share similar characteristics. If metals are incorporated into an orthosis or prosthesis, the choice of metal is determined by the needs and preferences of the particular patient.
Steel
The general term steel refers to any iron-based alloy material. Carbon alloys have carbon added to the composition of steel. The term alloy steel is used when other materials are included in the material manufacture. Alloy steels are further defined as low-alloy or high-alloy steels. Steels are strong, rigid, ductile, and durable, but their high density (weight) and susceptibility to corrosion are major disadvantages. Many different types of steel are available to meet various engineering needs. To assist in identifying the composition and type of material, the American Iron Steel Institute–Society of Automotive Engineers has established a four-digit numbering system. The first two digits in the number indicate the type of steel, and the last two digits identify the carbon content. For alloy steels, the first digit identifies the major alloy and the second digit indicates the percentage of the major alloying element.
The carbon content of steel is the major determinant of its ductility and yield strength characteristics. Yield strength is an offset measure in a stress-strain curve in which strain occurs without an increase in stress. Ductility is the property of a material to deform in the inelastic or plastic range. Low carbon content (0.05% to 0.10%) produces high ductility and a low yield strength.15 As the carbon concentration increases, yield strength increases and ductility is reduced. Heat treatments can alter the properties of carbon steel by increasing yield strength and reducing ductility. The mechanical properties of low-alloy steels fall between those of carbon steels and high-alloy steels. High strength/weight ratios are possible with the high-alloy steels, an important characteristic for repetitive loading situations. These types of steels are used for some orthotic and prosthetic joint components. High-alloy steels are not very resistant to corrosion and are often more difficult to fabricate.
Stainless steel is a steel alloy that contains 12% or more of chromium, a material that increases resistance to corrosion and oxidation. Chromium produces a light oxide film on the surface that deters deterioration of the base metal. Because durability and protection from corrosion are highly desirable, stainless steels are used extensively within orthotics and prosthetics to enhance longevity of devices. Two types of stainless steel, martensitic steel and ferretic steel, have chromium as the predominant alloying element, but martensitic steel is the only one used in orthotics and prosthetics because it can be hardened by heat treatment. Stainless steel is used for orthotic and prosthetic joints, support uprights, and band material.
Aluminum
Aluminum alloys are well suited for orthotics and prosthetics because of their high strength/weight ratio and resistance to corrosion. As with steels, the properties of aluminum depend on alloying compositions, heat treatments, and cold working. Wrought and cast are terms used to describe the two major types of aluminum alloys. Alloys are further subdivided into those that are heat treatable and those that are not. The low ductility and low strength of cast aluminum are ideal for prefabricated prosthetic components and in some assemblies for moving parts.
Wrought aluminum alloys are used in orthotics and prosthetics for structural purposes such as prosthetic pylons, orthotic uprights, and upper extremity devices. The high-compression bending stresses of lower extremity prosthetics are well suited to the use of wrought aluminum alloys.
Although aluminum alloys are very resistant to atmospheric and some chemical corrosion, the acids and alkalis in urine, perspiration, and other bodily fluids deteriorate the natural protective oxides on the material’s surface, making the aluminum susceptible to corrosion. To deter corrosion in aluminum and to resist abrasive wear, various hard coatings, such as anodic or oxide finishes, can be applied. Mechanical finishes, such as polishing, buffing, and sandblasting, offer attractive cosmetic appearances for devices.
Titanium and Magnesium
Components made of titanium alloys have become more prevalent in prosthetics but are rarely used in orthotics. Although titanium alloys are stronger than those of aluminum and have comparable strength to some steels, their density is 60% that of steel.16 Because prosthetic components made of titanium are lighter in weight than steel counterparts, they require less energy expenditure by the patient during use. Titanium alloys are also more resistant to corrosion than are aluminum and steel. It is important to note, however, that titanium alloys are often more difficult to machine and fabricate. Consequently, titanium is most often used in prefabricated prosthetic components, when strength and light weight are of concern. Titanium is also more expensive than aluminum and steel, which has been a limiting factor for its use.
Magnesium alloys are lighter than those of aluminum and titanium, are corrosion resistant, and have a lower modulus of elasticity than does aluminum. The modulus of elasticity (Young’s modulus) is defined as the ratio of unit stress to unit strain in a stress-strain curve’s elastic range; materials with low modulus values are associated with lower rates of fatigue under conditions of repeated stress. Although some of these features are promising, magnesium alloys have not yet been widely used in orthoses and prostheses.
Wood
Wood possesses many desirable characteristics for use in prosthetics. Its wide availability, strength, light weight, and ability to be shaped easily have continued to be of benefit in prosthetic socket and component construction, even with the introduction of thermoplastics. The wood used in prosthetics must be properly cured, free of knots, and relatively strong. Yellow poplar, willow, basswood (linden), and balsa are most commonly used. Hardwoods have been reserved for prosthetic applications in which structural strength is essential, most often in certain types of prosthetic feet or as reinforcement for knee units. The keel prosthetic foot is fabricated of maple and hickory. The solid-ankle, cushioned-heel prosthetic foot has a hardwood keel that is bolted to the prosthetic shank, creating a solid structural unit for standing and ambulation.
Plastics and Composites
One of the most important production-related characteristics of an orthotic or prosthetic material is its ability to be molded over a positive model. Because plastics can be readily formed, they are a very popular, widely used material for orthoses and prostheses. Plastics are grouped into two categories: thermoplastics and thermosetting materials.17–19
Thermoplastics
Thermoplastic materials are formable when they are heated but become rigid after they have cooled. Thermoplastics are classified as either low-temperature or high-temperature materials, depending on the temperature range at which they become malleable. Low-temperature thermoplastics become moldable at temperatures less than 149°C and can often be molded directly on the patient’s limb, whereas high-temperature materials require heating to much higher temperatures and must be molded over a positive model of the patient’s limb.18 One advantage of thermoplastic materials is that they can be reheated and shaped multiple times, making possible minor adjustments of an orthosis or prosthesis during fittings. Thermoplastics are the material of choice for “shell” designs in which structural strength is required. Some of the more popular materials used are acrylic, copolymer, polyethylene, polypropylene, polystyrene, and a variety of vinyls.
Certain low-temperature thermoplastics, those moldable at temperatures less than 80°C, can be applied and shaped directly to the body. Some of the most commonly available materials include Kydex (Kleerdex, Aiken, S.C.), Orthoplast (Johnson & Johnson, Raynham, Mass.), and Polysar (Bayer, Pittsburgh, Pa.). These materials are most often reserved for orthotic devices that are designed to provide temporary support and protection. Their susceptibility to repetitive stress, high loads, and temperature changes usually limits their use to spinal and upper extremity orthoses. Because these devices are molded directly on the patient, no casting is necessary, and the time required from measurement to finished product is greatly reduced. Another important convenience of low-temperature thermoplastic materials is that no special equipment is required; hot water heated in an electric frying pan, a heat gun, and sharp scissors are all that are necessary to produce a functional splint or orthosis.
High-temperature plastics are frequently used in the production of orthotics and prosthetics. The most commonly used materials include polyethylene, polypropylene, polycarbonate, acrylic, acrylonitrile butadiene styrene, acrylics, polyvinyl acetate, polyvinyl chloride, and polyvinyl alcohol.
Polypropylene is a rigid plastic material that is relatively inexpensive, lightweight, and easy to thermoform. Polypropylenes, which can be further characterized as homopolymers or copolymers, are one of the most widely used plastics in orthotics. The material has a white, opaque color and is available in sheets of various thicknesses, from 1 mm to 1 cm. Polypropylene is impact resistant and can endure several million cycles of repetitive flexes. This attribute has been extremely useful in orthotics for hinge joints and spring assists in AFOs. The material is, however, susceptible to ultraviolet light and extreme cold and is sensitive to scratches and nicks. In prosthetics, the light weight of polypropylene makes it ideal for components such as sockets, pelvic bands, hip joints, or knee joints. Polypropylene is commonly used for prefabricated AFOs and preformed modular orthotic systems.
The long fatigue life of polyethylene during repeated loading situations makes this material suitable for a number of orthotic and prosthetic applications. Prosthetic sockets, orthotic hinge joint components, and compression shells for clamshell design orthoses are common uses of polyethylene plastics. Several densities of polyethylene are available from various manufacturers. Low-density polyethylene is used for upper extremity and spinal orthoses under the trade names Vitrathene (Stanley Smith & Co, Ltd, Isleworth, U.K.) and Streifen (FG Streifeneder KG, Munich, Germany). High-density polyethylene, Subortholen (Wilhelm Julius Teufel GmbH, Stuttgart, Germany), is used for spinal and lower extremity orthoses. The ultra-high-density polyethylenes such as Ortholen (Wilhelm Julius Teufel) are used principally for lower extremity orthoses.
Thermoforming
Thermoforming is one of the most common techniques used in orthotic and prosthetic laboratories to fabricate the “user” interface components of a device (e.g., prosthetic socket, AFO). The process of thermoforming entails heating a sheet of thermoplastic material in an oven until it has reached its “plastic” state (i.e., ability to distort) and then forcing the material over a prescribed shape (i.e., positive mold) under pressure until it has cooled. Negative air pressure or vacuum is the typical method used to apply pressure and form the plastic over the mold, hence the terms “vacuum-form” are also used to describe the process. Once the plastic has cooled and returned to its solid state, the perimeter of the formed components is determined and trimmed out. The edges of the plastic are then finished on specialized grinding machines that have a diverse set of abrasive sanding and buffing cone options that are used to achieve a high-polish smoothed edge.
Thermosetting Materials
Thermosets are plastics that are applied over a positive model in liquid form and then chemically “cured” to solidify and maintain a desired shape. To enhance their structural properties, thermosets are often impregnated into various fabrics by a process of lamination. Although this group of plastics has inherent structural stability, their rigidity precludes modification by heat molding; their shape can only be changed by grinding. Thermosetting plastics cannot be reheated without destroying their physical properties. Some of the most common thermoset resins used to produce rigid orthoses are acrylic, polyester, and epoxy. Because acrylic resins are strong, lightweight, and somewhat pliable, they offer a different set of characteristics than those of polyester resins. With lamination, acrylic resin can create a thin but strong structural wall for a prosthetic socket or component of an orthosis. If frequent adjustments are anticipated, however, thermoforming plastics are chosen instead because thermoset cannot be heated to make adjustments to their shape.
Composites
Composites are the combination of two or more materials with distinctly different physical or chemical properties that together produce a material with enhanced performance characteristics relative to their material properties as a single substance. Numerous types of composites exist under this broad descriptive term, ranging from natural materials such as wood to manmade materials such as concrete. This chapter focuses on the combination of fibers and matrices associated with thermosetting plastics.
Fiber-reinforced plastics (FRPs), also referred to as composites, have revolutionized orthotics and prosthetics, primarily because they can be engineered to have mechanical properties with strength characteristics optimized for specific types of loading situations, thereby greatly improving the functionality of many types of orthoses and prostheses. The mechanical properties of reinforcement fibers, for the most part, dictate the mechanical properties of a composite through the orientation and position of the fibers. In general, FRPs offer high strength and stiffness qualities yet are also capable of incurring compressive or flexural stresses. Plastics can also be reinforced with other fillers, particulates, and short or chopped fibers, but FRPs are most widely used. This section focuses on fiber reinforcement for thermosets.
Polymer composites are made of a binder material (i.e., resin), referred to as the matrix, which is reinforced with fibers to improve strength and stiffness. The matrix of a composite encapsulates the fibers to maintain their desired orientation and position and ensure that load sharing of fibers is well distributed through the material. The volume fraction of resin-to-fiber is an important determinant of the mechanical properties of a composite and its performance. In general, the volume of fiber should be higher than the volume of resin matrix. A fiber volume fraction that approaches 90.7% with a matrix volume fraction of 9.3% is considered an ideal ratio.20
By comparison, the fibers in composite materials are stronger than the matrix material and can handle stresses applied to them better than the weaker matrix material. Typical fiber reinforcements used in polymer composites are fiberglass, carbon/graphite, and Kevlar aramid fibers (DuPont, Wilmington, Del.). Grades of different fiberglass include E, C, and S glass, which stand for electrical (E), chemical (C), and strength (S), respectively. S glass has a higher tensile strength than E and C glass. Carbon fiber is widely used in orthoses and prostheses having strength properties greater than steel while also being lightweight and very stiff. Carbon fiber has superior stiffness properties in both compression and tension but, because it has relatively low impact strength, it is often combined with other reinforcement fibers like fiberglass or Kevlar to improve its performance. Aramid fibers (e.g., Kevlar) have tensile strength properties that are five times greater than steel for the same weight.
Laminar composites or laminates are fabricated out of “continuous fibers” that may extend the length of a given part and are one of the most common FRPs used in orthotics and prosthetics. Continuous fiber reinforcements can be woven into the form of a fabric in many different weave patterns. By combining different types of fiber fabrics (e.g., carbon graphite, Kevlar) and stacking these plies into layers, the resultant laminate can possess properties for particular modes of loading. When engineering laminates for orthoses and prostheses, the practitioner or technician must understand the manner in which loads will be transmitted through a device so that appropriate layering and orientation of plies will be incorporated into the composite to meet its functional performance duties. A laminate code system is used to describe the direction and ply layer with the longer dimension of the laminate serving as the x-axis and the width serving as the y-axis. A fiber orientation angle is used to describe the orientation of plies with respect to the x-axis, which is designated as 0°. A laminate code describes the sequencing of each layer from top to bottom. An example of a laminate code where the top ply is 0° followed by subsequent plies oriented respectively at 45°, 90°, 45°, with the bottom ply at 0° would be written as follows: [0/45/90]2. Brackets define the code’s beginning and end, and the subscript indicates the adjacent plies are oriented the same, which is essentially one half of the ply description. The purpose of having a variety of different fiber orientation angles in composite laminates is that the load can be carried through the fibers in a number of different positions. For example, if the fibers were only oriented in one direction, then structural loading of the material at a different angle could result in a failure. Therefore multidirectional laminates have the capacity to endure loads in an orthotic and prosthetic device in variety of positions.
Processing Technologies and Composite Fabrication
Orthotic and prosthetic devices often are a compilation of custom-molded interface shell components combined with additional premanufactured parts (e.g., prosthetic foot, pylon). Practitioners and their technical staff have the capability to fabricate the human interface portions of a device within their laboratories, although the processes and equipment in their labs are limited to only a few techniques. Manufacturers on the other hand have the capacity to consider a much wider variety of processing techniques for mass producing composite parts and thus take advantage of processing technologies that can maximize the performance potential of the material.
The most common method for processing FPR composites in orthotic prosthetic laboratories is a contact molding technique called a “vacuum bag” lamination. The technique is relatively simple and does not require any specialized equipment. A flexible membrane bag of plastic is tightly stretched over the positive mold part and sealed; then negative air pressure (i.e., vacuum) is applied between the bag and the mold, drawing the bag tightly to the surface of the mold. A hand layup of fiber cloth is placed on the mold in a predetermined manner with regard to the orientation and position of the fibers with an understanding of how loads will be transmitted through the structure when it is incorporated into a device. After the desired layup of fiber and cloth is achieved, a second flexible membrane bag is pulled over the mold, creating an enclosure that can accommodate the wet liquid resin (matrix) part of the composite. After the resin is poured into an opening of the second bag and sealed, vacuum inside the enclosure draws the outer bag toward the mold, pressing the resin through the fiber in a uniform manner to create a thin-walled structure of composite that has a high fiber-to-resin ratio. If the atmospheric pressure that presses on the outside bag is not adequate to achieve the desired fiber-to-resin ratio, then the vacuum bag mold construct can be placed into an autoclave to create higher pressures on the external bag. Because most orthotic prosthetic laboratories do not have autoclaves for such procedures, central fabrication laboratories equipped with such equipment are being used to a greater extent to maximize the benefits of pressure processing in composites.
Foamed Plastics
Foamed plastics can be used as a protective interface between the orthotic or prosthetic and the skin, especially over areas that are vulnerable to pressure, such as bony prominences. Foamed plastics are grouped into two classes: open and closed cell. Cells are created in rubber or polymers in a high-pressure gassing process.18 The microcell structure allows the foamed plastic material to be displaced in several planes, which is an ideal physical property for the reduction of shear forces. In an open-cell foam, the cells are interrelated (as in a kitchen sponge); in a closed-cell foam, the cells are separate from each other. Because closed-cell foams are impervious to liquids, they are less likely to absorb body fluids such as perspiration or urine; however, they do act as insulators and can be hot when worn for extended periods.
An orthopedic grade of polyethylene foam was introduced in the 1960s by a British subsidiary of the Union Carbide Company.21 These closed-cell foams are available in a wide array of durometer hardness. (Durometer refers to a spring indenture post instrument that is used to measure the resistance to the compression/hardness of a material.) Polyethylene foams are commercially available under trade names such as Plastazote (Hackettstown, N.J.), Pe-Lite, Evazote (Bakelite Xylonite Ltd., Croydon, U.K.), and Aliplast (Alimed Inc., Dedham, Mass.). Various polyethylene foams are used in the manufacture of soft and rigid orthoses, depending on the density of the material. Plastazote is a low-temperature, heat-formable foam that has been used successfully in the treatment and prevention of neuropathic foot lesions.22–25 Its light weight and forgiving quality to bony prominences make it a desirable interface for the insensate foot. See Hertzman22 for a complete review of the use of Plastazote in lower limb orthotics and prosthetics.
Closed-cell foams are also made with synthetic rubber or polychloroprene. Neoprene is available in various densities, making the low-durometer versions suitable as liners for orthoses, whereas the firmer materials are used for posts or soling for shoes. Spenco (Spenco Medical Corp., Waco, Texas) is a microcellular neoprene foam that reduces shear forces to the foot’s plantar surface and the occurrence of foot blisters in athletes.26 The nylon (polyamide)-covered neoprene acts as a shock absorber while also reducing friction on the foot’s plantar surface.21 Although few of these materials are heat moldable, most can be conformed without difficulty to the shallow contours of foot orthoses. Lynco (Apex Foot Health Industries, Teaneck, N.J.) is an open-cell neoprene foam that dissipates heat more efficiently than its closed-cell cousin; however, it does not attenuate shock as well as Spenco.21
Polyurethane open-cell foams are alternatives for top covers for foot orthoses. They provide good shock absorption and dissipate heat well. Some of the commercially available open-cell polyurethane foams include Poron (Rogers Corporation, Rogers, Conn.), PPT (Professional Protective Technology, Deer Park, N.Y.), and Vylite (Steins Foot Specialties, Newark, N.J.).
Several studies that compare materials used to fabricate orthoses have been conducted.27–33 In 1982, Campbell and colleagues27 conducted compression tests on 31 materials to determine their suitability for insoles in shoes. Materials were classified according to stiffness into the categories “very stiff,” “moderately deformable,” and “highly deformable.” The moderately deformable group of plastics, which included 19 of the tested foamed plastics, was deemed the most beneficial as an insole material. Campbell and colleagues27 concluded that these materials could relieve stress from bony prominences and transfer the loads to the adjacent soft tissues more effectively than could the very stiff or highly deformable materials. Studies evaluating shoe insole materials also report them to be effective at attenuating shock during walking in various ways.33
Viscoelastic Polymers
A viscoelastic solid is a material that possesses the characteristics of stress relaxation and creep. Stress relaxation occurs when a material that is subjected to a constant deformation requires a decreasing load with time to maintain a steady state.34 Creep refers to the increase in deformation with time to a steady state as a constant load is applied.34 Sorbothane (Sorbothane, Inc., Kent, Ohio), widely used as an insole material, is made of a noncellular polyurethane derivative that possesses good shock-attenuating characteristics.34 Viscolas (Viscolas Corp., Soddy Daisy, Tenn.), another type of viscoelastic solid, has been found to attenuate skeletal shock at heel strike in the tibia to half the normal load.15,35 Two other viscoelastic polymers used to fabricate orthotic prosthetic components are Viscolite (Polymer Dynamics, Inc., Allentown, Pa.) and PQ (Riecken’s Orthotic Laboratories, Evansville, Ind.).
Prescription guidelines
The formulation of a prescription for an orthosis or prosthesis greatly influences the potential functional outcome for the patient. It is critical that rehabilitation objectives and design criteria be carefully considered. Physicians, therapists, orthotists, and prosthetists who are involved in developing a prescription for an orthotic or prosthetic device must have a sound understanding of orthotics and prosthetics to be successful in effectively treating patients with these devices.
Assessment of functional deficit includes a thorough evaluation of the patient’s present physical status, including muscle strength testing, range of motion measures, and documentation of other physical impairments that would affect the fit or performance of the device. Equally important to the physical examination is the consideration of any individual needs of the patient and an understanding of how the treatment will affect daily activities. To increase the success of treatment, the patient and other rehabilitation team members must reach a consensus on the type of device and the associated training and education required for optimal functional outcome.
Orthotic Prescription
The Committee on Prosthetics and Orthotics of the American Academy of Orthopaedic Surgeons developed a technical analysis form to standardize the process of patient evaluation. This evaluation protocol documents the biomechanical deficits of the patient and provides the basic information needed for orthotic prescription formulation. This systematic approach has two major objectives: to define the anatomical segments that the orthosis will encompass and to accurately describe the biomechanical controls needed for treatment. The underlying principle of this assessment is that orthoses should be designed to control only those movements considered abnormal while permitting free motion in anatomical segments that are not impaired.
Technical analysis forms were developed for three general regions of the body: the upper limb, lower limb, and spine. The forms are four pages long with the same basic approach for formulating an orthotic prescription. The first page has sections for recording general patient information and noting major physical impairments (Figure 6-1). The major impairment section characterizes any functional limitations, such as skeletal structure, sensation, or joint contracture. This information provides an overview of the patient’s clinical presentation.
< div class='tao-gold-member'>
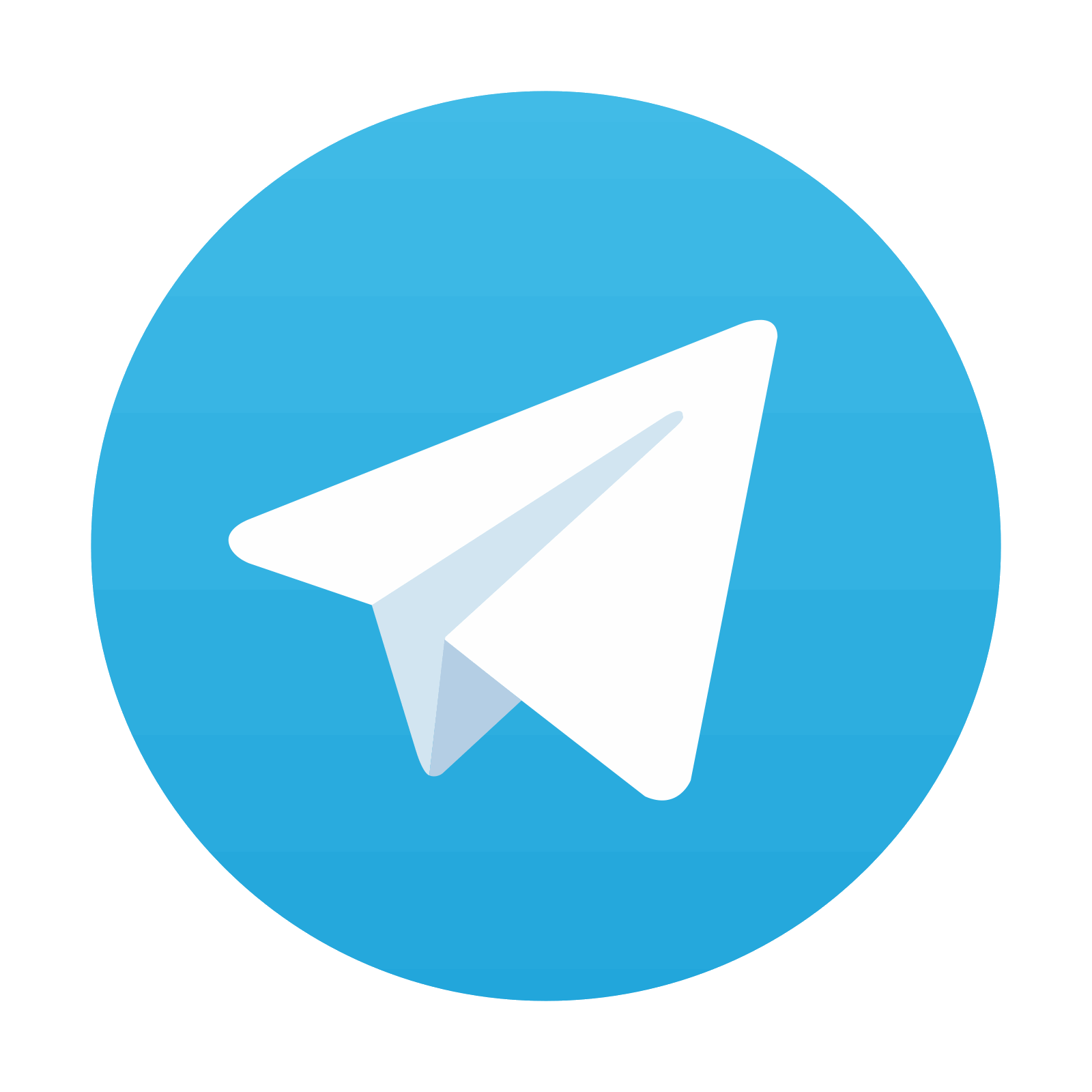
Stay updated, free articles. Join our Telegram channel
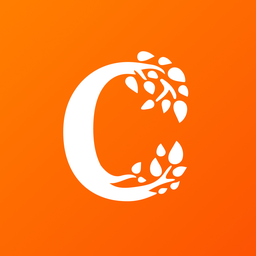
Full access? Get Clinical Tree
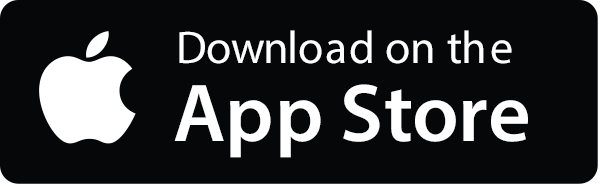
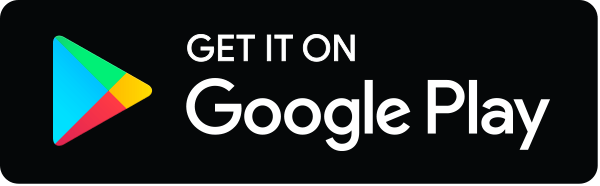