8 Key Points 1. Clinical management of SCI in the ICU is centered on the identification, prevention, and treatment of secondary insults, with a specific emphasis on maintaining adequate perfusion and oxygenation of injured nervous tissue. 2. Hemodynamic instability and ventilatory failure are common after SCI, especially in cervical and high thoracic injuries, and can occur in a delayed fashion. 3. The ICU provides the optimal environment for early detection and treatment of cardiovascular instability and respiratory failure in patients with SCI and can potentially reduce complications and improve outcome after SCI. The treatment of spinal cord injury (SCI) has evolved significantly over the past decades. Treatment of acute SCI in the intensive care unit (ICU) (Fig. 8.1) can provide several benefits, such as monitoring and management of cardiopulmonary dysfunction. Although some centers have created units dedicated solely to the treatment of patients with SCI,1–4 this type of specialized care has not been universally accepted, and there is still a wide range of management strategies within regions and even within specific institutions. Specifically, the question of whether patients with acute SCI benefit from care in the ICU has not been fully answered. This chapter describes the scientific rationale behind ICU management of SCI and the main issues specific to SCI that can be addressed in an intensive care setting. The pathophysiology of SCI is generally separated into primary and secondary injury mechanisms. Primary injury occurs at the time of trauma, and common mechanisms include mechanical compression, laceration, shear, and penetrating injury. Although these processes are heterogeneous, they all result from external mechanical forces transferred to the spinal cord tissue. The primary injury event initiates a cascade of secondary injury mechanisms, including (1) vascular injury and compromise that can lead to loss of auto-regulation, vasospasm, thrombosis, and hemorrhage; (2) electrolyte imbalances, cellular integrity loss, energy metabolism failure, and edema; (3) neurotransmitter accumulation and toxicity, free radical buildup, and lipid peroxidation; and (4) delayed cell death or apoptosis.5–7 Fig. 8.1 Typical intensive care unit room set up with multimodal monitoring at Sunnybrook Health Sciences Centre, Toronto, Canada. The molecular mechanisms involved in this secondary injury cascade are similar to those involved in other types of neurological injury, such as traumatic brain injury (TBI) and acute ischemic stroke. These various pathways of cellular injury have been the focus of extensive preclinical work on neuroprotection for SCI. However, at present, clinical trials targeting secondary injuries have shown only limited benefit in patients. An additional critical aspect of secondary injury after SCI is avoidance of secondary insults. Secondary insults are clinical events that would otherwise be well tolerated but exacerbate damage to vulnerable tissue that has been “primed” by the initial injury. Examples of secondary insults frequently encountered early in SCI that can be identified and managed in an intensive care setting are hypotension, hypoxia, fever, and hyperglycemia. Hypotension and hypoxia result in decreased substrate delivery of oxygen and glucose to the injured cord, whereas pyrexia may further increase metabolic demand, and hyperglycemia may exacerbate ongoing injury mechanisms. The absolute primacy of the “ABCs” of trauma patients with confirmed or suspected SCI cannot be overemphasized. Adequate perfusion and oxygenation of injured nervous tissue are essential to optimize recovery. Even brief periods of hypoperfusion and hypoxia can trigger secondary injury cascades, which increase morbidity and mortality and decrease chances of neurological recovery.7 Current clinical approaches to the management of SCI in the ICU are centered on these concepts of primary and secondary SCI. Likewise, the identification, prevention, and treatment of secondary insults are the principal focus of neurointensive care management for patients with acute SCI. Studies in animal models of SCI suggest ischemia is a common denominator or end-result of continuing neurological compromise following the primary injury.8 At the spinal cord level, direct injury to the microcirculation results in vasospasm and loss of autoregulation, leading to alteration in spinal cord blood flow.6 At the systemic level, acute SCI is often associated with hemodynamic instability. This is particularly more common and pronounced in cervical and complete SCI. Hypotension in the setting of SCI and trauma can have multiple etiologies: (1) Loss of sympathetic vasomotor tone results in a decrease in systemic vascular resistance and pooling of blood in the peripheral vasculature. (2) Interruption of sympathetic input to the thoracic region results in unopposed parasympathetic outflow to the cardiac fibers, which leads to arrhythmias. The most commonly seen arrhythmia is bradycardia, although supraventricular and ventricular tachycardia can also occur.9 Arrhythmias tend to be more common in the first 2 weeks following injury and more pronounced in more severe injuries.9 (3) Since a significant proportion of SCI patients also have multisystem injuries, hypovolemia from internal bleeding can be a significant contributor to hypotension. Typically, hypotension related to hypovolemia is associated with tachycardia, whereas hypotension seen with severe SCI is often coupled with bradycardia. Systemic hypotension in the setting of SCI, with coincidental loss of autoregulatory function in the microcirculation, further compromises local spinal cord perfusion and blood flow and exacerbates spinal cord ischemia.6,8 The first line of treatment is volume resuscitation using 1 to 2 L of crystalloids. Volume expansion alone may not be sufficient to restore normotension if the increase of venous return is not matched by an accompanying increase in cardiac output; the addition of vasopressor pharmacological agents may be required. The choice of vasopressor should be an agent that has both α- and β-adrenergic actions, such as dopamine or norepinephrine. Dobutamine can help increase the cardiac output but also causes a decrease in systemic vascular resistance, which can compound the hemodynamic effect of SCI and is therefore less useful. Phenylephrine has a purely α-adrenergic effect and should be used with caution because the increase in cardiac afterload and lack of β-adrenergic response, especially in cervical SCI, can result in reflex bradycardia.10,11 Restoration of normal hemodynamic parameters is the primary goal and can be demonstrated by adequate urine output, resolution of systemic acidosis, and return of normal mentation in conscious patients. The absolute values for blood pressure and cardiac output that are required to achieve this will vary between patients. The appropriate end point necessary to achieve adequate spinal cord perfusion and blood flow is more difficult to define. There is good evidence from animal studies that hypotension contributes to spinal cord ischemia after injury and can worsen the initial insult and reduce the potential for neurological recovery.12 Similar evidence is lacking in human SCI. The effect of hypotension in human TBI has been studied comprehensively and inference from the TBI literature seems appropriate.13 Prospectively collected data from the Traumatic Coma Data Bank demonstrated that hypotension, defined as systolic blood pressure of less than 90 mmHg and hypoxia, defined as PaO2 below 60 mmHg, were independently associated with a significant increase in morbidity and mortality after severe TBI.13 A single episode of hypotension was associated with a 50% increase in mortality. Despite the lack of similar human studies in SCI, therapeutic interventions aimed at correcting hypotension and maintaining threshold levels of mean arterial pressure (MAP) to improve spinal cord perfusion have promising potential. Several reports of case series suggest that treatment of hypotension and resuscitation to maintain MAP at high-normal levels, 85 to 90 mmHg, may enhance neurological outcome after acute traumatic SCI.1–4,14,15 Vale et al.,3 in 1997, reported their experience with a nonrandomized, prospective pilot study in the assessment of aggressive medical resuscitation and blood pressure management in 77 consecutive ASCI patients. All patients were managed in the ICU with invasive monitoring, including pulmonary artery catheters and arterial lines, while blood pressure was optimized to maintain MAP above 85 mmHg for 7 days after injury. The average admission MAP for complete cervical SCI patients was 66 mmHg. Nine of 10 (90%) patients with complete cervical SCI required vasopressors after volume replacement, compared with 13 of 25 (52%) with incomplete cervical injuries, and 9 of 29 (31%) with thoracic injuries. At 1 year follow-up, three of 10 complete cervical SCI patients regained ambulatory capacity, and two patients regained bladder function. Incomplete cervical SCI patients fared better. Twenty-three regained ambulatory function at 12-month follow-up, only four of whom had initial examination scores consistent with walking. Twenty-two of 25 (88%) patients regained bladder control. Thirty-one of 35 (89%) cervical SCI patients and 27 of 29 (93%) thoracic level SCI patients were treated surgically. The results were independent of neurological function at admission and timing of surgery. The authors concluded that the enhanced neurological outcome identified in their series after acute SCI was the result of early and aggressive volume resuscitation and blood pressure augmentation and was cumulative to and/or distinct from any potential benefit provided by surgery. The results of this study and five other case series1,2,4,14,15 showing similar trends have led to the establishment of blood pressure treatment guidelines, with the understanding that class I evidence from randomized controlled trials on the subject are unlikely to be obtained in the future for acute SCI.10 Correction of hypotension (systolic BP < 90 mmHg) is offered as a strong treatment option,10 whereas class III evidence from the literature suggests that maintenance of MAP at 85 to 90 mmHg after ASCI for 7 days is safe and may improve spinal cord perfusion and, ultimately, neurological outcome.3,10 Respiratory complications are a major cause of mortality and morbidity in patients with SCI.16 Respiratory failure is common in SCI patients and occurs more often in cervical and complete SCI.17 The two phases of respiration, inspiration and expiration, are both affected after SCI. The process of inspiration requires the contraction of the diaphragm and the internal intercostals, which allows chest expansion. Accessory muscles can be recruited for increased respiratory activity. The C3-5 spinal cord segments and corresponding nerve roots innervate the diaphragm, and injuries above the C3 level result in apnea and the need for immediate ventilatory support. In the acute phase, flaccid paralysis of the intercostals leads to an overall decrease in size of the thoracic cavity when the diaphragm contracts. This results in a significantly diminished forced vital capacity to ∼ 70% of normal.18 Expiration is principally a passive process but can be augmented by contraction of the abdominal musculature. The loss of abdominal wall function in SCI leads to a similar decrease in expiratory force with accompanying impairment in the ability to cough and clear secretions.18 With time, the respiratory musculature becomes more spastic and the chest wall more rigid, with a resulting improvement in ventilatory function. Most of this improvement is seen during inspiration, with a return of forced vital capacity and maximal inspiratory force to ∼ 60% of baseline.18 The loss of sympathetic tone in the acute phase also results in increased bronchial tone from unopposed cholinergic bronchoconstriction.18 In addition, mucus production is increased while ciliary muscle activity is decreased. All of these respiratory changes can lead to relative hypoxemia and exacerbate spinal cord ischemia after acute SCI. It is therefore paramount to identify patients at risk for respiratory failure and complications. The altered mechanics of respiration lead to a pattern of shallow breaths, which can be initially compensated by an increase in respiratory rate. However, rapid shallow breathing does not adequately move the air out of the dead space zones, which do not participate in gas exchange, and also promotes atelectasis. The overall result is a higher work of breathing and decreased gas exchange, superimposed on diminished inspiratory force. Approximately one third of patients with cervical SCI will require endotracheal intubation and ventilatory support.19 Careful monitoring and some degree of judgment are necessary to identify patients before respiratory decompensation occurs. A decrease in vital capacity < 1 L as well as rising respiratory rate and arterial PCO2 are good indicators of impending respiratory failure. It is best to use a proactive approach of early intubation under controlled circumstances before the patient’s condition becomes an emergency to avoid devastating consequences, such as prolonged hypoxia, which can exacerbate secondary injury to the spinal cord. In the setting of a cervical spine injury, orotracheal intubation can be performed safely by experienced practitioners using manual in-line traction.20 In their retrospective review of 186 patients with low cervical SCI (C5-T1), Hassid et al. reported 58% complete SCI, with an overall intubation rate of 68%, tracheostomy rate of 69%, and mortality rate of 15%. Patients with incomplete SCI required intubation less frequently, at 38%, but still had a high proportion of tracheostomy (50%) for intractable pulmonary failure.16 Their data emphasize the need for rapid and comprehensive assessment of respiratory status in all patients with SCI, especially in cervical levels. Lu et al. reported eight cases of mid-low cervical SCI who developed delayed apnea with catastrophic consequences.17 Associated findings included the presence of diffuse, extensive cord lesions, respiratory distress, and bradycardia with or without associated hypotension, even when transient and self-limited. Sleep was also found to be an at-risk period of time in five of the eight cases. Evidence from animal models has attributed this type of delayed clinical deterioration and respiratory failure to progressively expanding cord lesion.6 In summary, patients with cervical SCI often present initially without any evidence of respiratory compromise but have a high risk of developing respiratory failure during the initial course of their clinical progression. Patients with thoracic SCI are also more at risk for respiratory complications than patients with lower injuries. Cotton et al. reviewed 11,080 patients with thoracic and lumbar spinal injuries, with 596 having a thoracic SCI. Respiratory complication rates were higher in high thoracic SCI (T1-6, 51%), than in lower thoracic injuries (T7-12, 34.5%) and thoracic fractures without SCI (27.5%).21 The need for intubation, risk of pneumonia, and mortality were all more prevalent in the higher thoracic SCI group. Aside from altered breathing mechanics associated with denervation of the upper thoracic segments, a potential explanation for the increased risk of respiratory complications in this group is the higher Injury Severity Score (ISS). A greater amount of force is usually required to produce significant damage to the thoracic spine because of the increased stiffness provided by the supporting rib cage and costotransverse articulations and ligaments. This is associated with greater risk of injury to the thoracic cavity, such as flail chest wall segments, pulmonary contusions, pneumo-/hemothoraces, and cardiac injuries. A significant proportion of respiratory morbidity and mortality in SCI is attributable to pneumonia. Ventilator-associated pneumonia (VAP) is a direct consequence of intubation and prolonged ventilation. The risk of developing VAP increases by 1 to 3% per day of intubation.22 Streptococcus pneumoniae and Haemophilus influenzae are the pathognomonic organisms in the early period (< 4 days), whereas gram-negative species and Staphylococcus aureus are the usual causative agents in later periods. Overall mortality of VAP has been reported as 27%, and as high as 43% when due to Pseudomonas aeruginosa.23 Diagnosis of VAP is challenging because many associated radiographic findings are nonspecific, and laboratory abnormalities are common in critically ill patients. Increasing amounts of secretions may be an indicator of early pneumonia, but many patients have copious secretions without an associated infection. Empirical treatment without clear evidence of actual infection can lead to the selection of antibiotic-resistant organisms, whereas delayed treatment can have serious consequences. Recommendations from the American College of Chest Physicians for the diagnosis of VAP include temperature > 38°C or < 36°C, leukocytosis or leukopenia, purulent secretions, and hypoxemia. These criteria, in combination with an abnormal chest radiograph showing air bronchograms or alveolar infiltrates, warrant starting antibiotic therapy tailored to organisms grown from tracheal aspirates.11 Respiratory mechanics improve steadily after the acute period, allowing weaning from ventilatory support, especially if the level of injury is at C4 or below.24 The average period of ventilator dependence is strongly related to the level of injury: 65 days for patients with C1-4 levels, 22 days in patients with C5-8 levels, and 12 days for patients with thoracic injuries.25 Parameters that suggest a patient is ready for weaning include rise in forced vital capacity, FIO2 requirement < 50%, minute ventilation < 10 L, and resolution of any respiratory complications, such as pneumonia.11 The majority of patients take 2 weeks or more before they are ready for weaning.19 Early mobilization helps the process of weaning in most patients. However, it has been shown that quadriplegic patients have better ventilatory mechanics in the supine position than in the upright position.26 Flaccid abdominal musculature allows the abdominal contents to descend in the upright position, which overdistends the diaphragm and decreases its contraction efficiency. This may be helped with the use of abdominal binders.27 Changes in ventilation mode can also assist the weaning process; they include T-piece trials, continuous positive airway support, and pressure support mode.28 Each method has specific advantages, and, although it is not clear which is superior, pressure support ventilation has become increasingly prevalent because it allows for slow titration of the amount of ventilatory support.28 The use of tracheostomy offers clear advantages in patients with SCI who require prolonged ventilation: (1) The amount of dead space and resistance across the smaller airway is decreased. (2) It permits easier suctioning of secretions (pulmonary toileting). (3) It allows for periods of spontaneous breathing to alternate with mechanical support without manipulating the airway. (4) It is more comfortable for the patient. (5) It may decrease the incidence of pneumonia. For patients who require an anterior cervical surgical stabilization, it is generally recommended to wait 1 to 2 weeks between the two procedures to avoid contamination of the surgical wound by respiratory secretions. Cameron et al. recently reported their experience before and after implementation of an interdisciplinary tracheostomy team supervising SCI patients with tracheostomies.29 They found a significant decrease in median length of stay, decrease in median duration of cannulation, increase in the use of speaking valve, and decrease in time to valve trial, and no tracheostomy-related emergency, compared with two events in the preimplementation period. The incidence of cervical spine injury in polytrauma patients is relatively small, ∼ 1 to 3%. Even so, the potential for devastating consequences from neurological compromise related to a missed diagnosis requires that all trauma victims be managed with the expectation that a spinal injury is present. This implies that the cervical, thoracic, and lumbar spines have to be “cleared” during the hospital stay. Prolonged supine immobilization has significant morbidity, including pressure sores, raised intracranial pressure (ICP), airway and respiratory complications, difficulty with central venous access, gastric reflux and aspiration, and limitations in physiotherapy care. Most of these complications can occur quickly after admission and can progress rapidly after 48 to 72 hours. Furthermore, there is some evidence that rigid collars do not restrict the displacement of unstable cervical injuries.30 The presence of severe TBI is associated with an increased risk of cervical spine injury. A Glasgow Coma Scale score of < 8 has been shown to be associated with a 50% increase in incidence of cervical spine injury.31 Moreover, the management of many polytrauma patients requires sedation for other reasons such as ventilatory care or the need for repeat/multiple surgeries, and clinical assessment may therefore be impossible for prolonged periods. In the unconscious or unexaminable patient, imaging modalities occupy a central role in clearing the spine. The overall complications related to prolonged immobilization and rigid cervical collar are not insignificant and have to be balanced against the small risk of an undetected or unstable ligamentous injury missed by radiological modalities. There is currently no standardized approach in the clearance of the cervical spine in unconscious and intubated patients,31 which reflects the difficulty this problem poses in the ICU. Several imaging modalities have been used to clear the cervical spine. Cross-table lateral plain radiographs require the visualization of the entire cervical spine, including the craniocervical junction and the cervicothoracic junction. When these images are anatomically and technically adequate, their radiographic sensitivity in detecting cervical injuries is ∼ 80%.31 It is estimated that a single lateral plain radiograph will miss ∼ 15% of injuries, and typically 50% of these films are inadequate.31 The addition of an open-mouth view for odontoid visualization and an anteroposterior view to better asses the facet joints and rotation abnormalities increases the sensitivity to ∼ 90%. The odontoid view is often obscured in unconscious patients by artifact from endotracheal and gastric tubes and cervical collars, and ∼ 25 to 50% of films are inadequate for proper interpretation. Computed tomography (CT) has evolved rapidly in recent years, and it is difficult to interpret older studies of its efficacy in spinal injury detection. Improvements like thinner axial slice acquisition (1.5 to 2 mm), sagittal and coronal reconstructions, and digital viewing may all have helped in bringing CT to the forefront of imaging modalities for spinal clearance. Directed CT scanning involves scanning of only the nonvisualized or suspicious areas identified on plain films. Most studies show that the addition of directed CT detects an additional 10% of injuries not seen on plain films.31 CT scanning of the entire cervical spine detects significantly more injuries than plain films or directed scanning. Studies have shown a sensitivity approaching 100% for entire spine CT and improvement in detection of 10%.31 Magnetic resonance imaging (MRI) is the investigation of choice for spinal cord evaluation in SCI. However, its role in spinal clearance is less clear. MRI has a theoretical advantage over CT in detecting ligamentous injuries. MRI is extremely sensitive for soft tissue injuries, but the significance of many detected abnormalities is unknown. Most abnormalities severe enough to require surgical fusion can be detected by CT.32 Dynamic fluoroscopy involves passively manipulating the cervical spine under real-time imaging and has the advantage of demonstrating actual instability under stress. The main limitation of this method is the inability to obtain an anatomically adequate study, particularly at the cervicothoracic junction, which is missed in ∼ 40% of cases. There are also legitimate concerns regarding cervical manipulation in unconscious patients. In their comprehensive review of cervical spinal clearance, Morris and McCoy found 10 separate studies on dynamic fluoroscopy.31 Of a total of 887 patients, only 10 (0.9%) cervical injuries were detected by dynamic fluoroscopy, five (0.6%) of which required surgery, with an overall number of 177 that needed treatment.31 None of the studies included high-resolution CT of the entire cervical spine as part of their investigation. Spiteri et al. did a retrospective review of their clearance protocol over 10 years and found 87 patients with unstable injuries, 85 of which were identified by CT, with a sensitivity of 97.7% and a specificity of 100%.33 Of the two cases that were missed, dynamic screening detected one (sensitivity 98.8%, specificity 100%), and missed the other. Review of the second case confirmed that the abnormality, an atlantooccipital dislocation, was identifiable on CT. The authors concluded that high-resolution CT alone could be reliably used to clear the cervical spine and indicated that dynamic screening was removed from their routine clearance protocol. Patients with acute SCI frequently experience hypotension, arrhythmias, hypoxemia, airway compromise, and pulmonary dysfunction.3,16,17,28,34 Hemodynamic instability and ventilatory failure can occur in a delayed fashion in patients who appear stable early during their postinjury course. The general consensus that secondary insults in the form of hypotension and hypoxia can further compromise the injured cord, combined with the high incidence of associated multisystem injuries, provide a sound basis for admitting, monitoring, and treating SCI patients in an intensive care setting in the acute phase. A few clinical series have tried to determine whether patients with acute SCI benefit from care in the ICU. In 1984, Tator et al. reported their experience in the care of 144 patients treated in the Acute Spinal Cord Injury Clinic at Sunnybrook Medical Centre between 1974 and 1979 and compared their results to a previously published cohort of patients treated in the same area from 1948 and 1973.2 Some of their core principles included early referrals, rapid transfer time, and early admission to their specialized unit, as well as aggressive treatment of hypotension and respiratory failure. The median interval time from injury to admission was 4.9 hours, compared with 12 hours in the previous period. Neurological status was assessed in 95 patients, of whom 41 (43%) had neurological improvement, 52 (55%) did not change, and only two had neurological deterioration. Mortality rates were significantly lower, at 6.9%, compared with 14.0% in the earlier group, and the authors attributed this mainly to improved respiratory care. Length of stay in the ICU was also reduced by ∼ 50% for both complete and incomplete injuries. Levi et al. treated 50 patients with acute cervical SCI according to an aggressive protocol that included invasive hemodynamic monitoring with arterial lines and pulmonary artery catheters, and treatment with volume and vasopressors to maintain adequate cardiac output and MAP > 90 mmHg.1 Eight patients presented with SBP < 90 mmHg at admission, and 82% of patients required vasopressor support in addition to volume replacement to achieve the target MAP. Patients with complete injuries had 5.5 times the rate of vasopressor requirement compared with incomplete injuries. The authors reported improvement in neurological function in 40% of patients, whereas 42% remained unchanged, and the overall mortality was 18%. There was minimal morbidity with invasive hemodynamic monitoring. The authors concluded that hemodynamic monitoring in the intensive care setting allows early recognition of cardiac dysfunction and hemodynamic instability with a potential to reduce morbidity and mortality after SCI. The study from Vale et al.3 is described in an earlier section. Their group also reported a high incidence of vasopressor requirement in SCI patients, and improved neurological outcomes with invasive monitoring in the ICU and aggressive blood pressure treatment compared with previously published studies. The authors chose the high-normal target for MAP of 85 mmHg arbitrarily based on their previous experience with TBI patients, and a length of treatment of 7 days based on data from experimental SCI studies showing a period for maximal spinal cord edema lasting between 3 and 5 days. There were no complications related to the invasive hemodynamic monitoring. Casha and Christie recently did a comprehensive literature review of cardiopulmonary management after SCI.35 Their findings confirm that (1) the incidence of cardiorespiratory complications after acute SCI is high and warrants care in specialized monitoring units; (2) the period of greatest risk for hemodynamic instability ranges between 1 and 2 weeks, whereas respiratory failure requiring mechanical ventilation can last weeks; (3) a target MAP > 85 mmHg in the first week after acute SCI is recommended, although the evidence is weak; (4) high cervical and complete SCI are the strongest predictive risk factors for cardiopulmonary complications; and (5) atelectasis and copious secretions are frequent obstacles to ventilatory weaning, and there is weak evidence to support that chest physiotherapy and aggressive suctioning are useful in the prevention of further respiratory complications. The difficulty in finding objective, absolute targets and treatment thresholds for hemodynamic, respiratory, and metabolic parameters to optimize neurological recovery and prevent secondary injuries represents a unique challenge in SCI. In the treatment of TBI, new technologies have been applied with success to complement standard monitoring modalities in the ICU. These techniques allow for the measurement of cerebral physiological and metabolic parameters related to oxygen delivery, cerebral blood flow, and metabolism with the goal of improving the detection and management of secondary brain injury. They include jugular venous bulb oximetry, parenchymal brain tissue oxygen tension monitoring, cerebral microdialysis, and intraparenchymal cerebral blood flow (CBF) monitoring. Initial observational data suggest that these monitoring tools provide unique information that may help to individualize management in severe TBI.36 Multimodality neuromonitoring in TBI is generally achieved with the placement of intraparenchymal probes. This type of monitoring is not desirable in SCI; intraparenchymal probe placement in injured cord tissue, or even in nearby normal areas, carries too great a risk for further injury. Noninvasive techniques, such as imaging, or less invasive extramedullary and extradural or surface monitors could provide alternatives to this problem. We know little about the physiological changes that occur within the spinal cord in the early at-risk period after human SCI. A better understanding of these variables would help significantly, especially in the ICU, where rapid changes in clinical condition or shifts in therapeutic approach could be tailored based on the prevention of secondary injury. Concepts taken from the TBI field can be extrapolated to SCI. ICP monitoring is central to the management of patients with severe head injury. Elevated ICP is associated with increased mortality and worsened outcome.37 Despite the lack of large randomized trials examining the effect of ICP monitoring on outcome, ICP treatment remains a core component of the guidelines for severe head injury management. Direct intraparenchymal spinal cord pressure monitoring is not feasible in humans. In TBI, ventricular catheters provide a cheap, reliable way of monitoring ICP and have the added benefit of allowing cerebrospinal fluid drainage to treat rises in ICP. A similar technique can be applied for SCI. Lumbar subarachnoid catheters can be inserted and give a reading of the intrathecal pressure, which is closely related to local tissue pressure.38 Lumbar catheters are routinely used in neurosurgery and spinal surgery for the treatment of cerebrospinal fluid (CSF) leaks with minimal morbidity. Pressure readings can be obtained using standard transducers and will vary with the position of the catheter and patient. Readings could also be limited in the event that spinal cord swelling obstructs the normal flow of CSF along the neuraxis. In theory, spinal pressure monitoring could be useful in determining whether monitoring and CSF drainage help in neurological recovery or if changes occur after surgical spinal decompression. Through autoregulation, the normal cerebral vasculature maintains an adequate blood flow across a wide range of mean arterial blood pressure. The spinal cord and intracranial microcirculations are similar, including their ability for pressure auto-regulation. Local vascular alterations and systemic hypotension following SCI can potentiate the ischemic injury. Similarly, induced systemic hypertension may increase the amount of hemorrhagic necrosis. Therefore, the relationship between systemic blood pressure and spinal cord blood flow is of vital importance for the management of patients with SCI. CBF optimization is a foundation of TBI treatment.37 Unfortunately, bedside measurement of CBF is not easily or widely obtainable. The concept of cerebral perfusion pressure (CPP) has been used as a more easily applicable alternative and is defined as the difference between the MAP and the ICP: CPP = MAP − ICP. It is the driving force for CBF through the cerebral vascular resistance. Because episodes of hypotension and raised ICP are both associated with worsened outcome in TBI, it is not surprising to find that episodes of low CPP are also associated with poorer outcome.39 The concept of spinal perfusion has also been used in a similar fashion. The pressure conducted through the spinal cord microcirculation is the gradient between the spinal arterial inflow and the venous outflow pressures. The inflow pressure is directly dependent on the MAP. The venous outflow is more difficult to determine. However, as in the cerebral compartment, the venous pressure can be approximated to be close to, or slightly higher than, the surrounding intrathecal pressure to prevent collapse of the venous system.38 Again in this situation, a lumbar catheter would allow direct measurement of the CSF pressure and approximation of the venous outflow pressure. Spinal cord perfusion pressure could be calculated as MAP–intrathecal pressure. Therapies aimed at augmenting spinal cord perfusion pressure and blood flow can be applied in the ICU if appropriate measurements can be obtained. In TBI, it was initially postulated that induced hypertension to target CPP > 70 mmHg with volume expansion and vasopressor agents would improve outcome. There is now a growing body of evidence to suggest that routinely artificially maintaining CPP above such high levels may not be beneficial and carries a risk of severe extracerebral complications, such as acute respiratory distress syndrome (ARDS). Thus the guideline-recommended CPP threshold is to target a CPP between 60 and 70 mmHg and avoid levels below 50 mmHg.37 Such guidelines do not exit for SCI, mainly because normal and pathological parameters have not yet been established. The current recommended treatment option of maintaining MAP at 85 mmHg for 7 days is based on a single uncontrolled pilot study.3,10 In pathological states where pressure autoregulation is altered, treatment based solely on perfusion pressure can potentially lead to perfusion breakthrough and secondary injury in the form of hemorrhage and edema. Knowledge of local spinal cord blood flow and vascular resistance can facilitate treatment decisions by determining if pressure autoregulation is intact or impaired. Hypothetically, patients with intact pressure autoregulation could benefit from higher MAP parameters, whereas more cautious settings could be applied to those with impaired autoregulation. Finally, it is possible that augmenting spinal cord perfusion pressure by lowering intrathecal pressure instead of increasing MAP has a more substantial effect in improving spinal cord blood flow. The concept of CSF drainage to prevent spinal cord ischemia is well established in thoracoabdominal aneurysm repair, where segmental arterial feeders and aortic cross-clamping can put the spinal cord at risk of infarction.40 The mechanism involved is not completely understood but may be related to a relative decrease in venous pressure with CSF drainage, leading to an increased arteriovenous pressure gradient (perfusion pressure) beyond the control of autoregulatory mechanisms. The spinal cord venous pressure itself also appears to be important in spinal cord perfusion. It is closely related to the central venous pressure because the cerebrospinal venous system is valveless. Etz et al. recently reported a retrospective analysis of 20 cases of paraplegia after thoracoabdominal aortic aneurysm repair and found significantly higher mean central venous pressures in the early postoperative period compared with a control group.41 The same is true in TBI, where elevations in central venous pressure are often accompanied by rises in ICP. Knowledge of the venous outflow pressure, which could be approximated by measuring intrathecal pressure, and local spinal cord blood flow would be essential in individualizing and optimizing treatment in SCI. Spinal cord blood flow measurement has been obtained in the past in animal models with invasive techniques. Magnetic resonance technology using arterial spin labeling has recently been used successfully to image local blood flow in the mouse spinal cord with relatively good spatial resolution.42 Evolution of this technique to human SCI should be feasible and would yield new insight in microcirculatory changes after SCI and could potentially guide treatment. The ICU provides the ideal environment for this type of multimodal monitoring and blood-flow-targeted therapies. Brain tissue oxygen tension (PbtO2) monitoring is now routinely used in specialized neurocritical care centers for the treatment of TBI. An intraparenchymal oxygen electrode measures PbtO2 when placed in the white matter. Despite the growing clinical use of this new technology, the specific determinants of low PbtO2 following severe TBI remain poorly defined. There is some indication that PbtO2 is more closely related to CBF and arteriovenous oxygen tension difference than oxygen delivery and metabolism.43 Normal PbtO2 is > 20 mmHg; duration and depth of PbtO2 below 15 mmHg are associated with worsened outcome.44 Noninvasive tissue oxygen monitoring techniques do not currently exist. Near-infrared spectroscopy has been used to measure brain tissue oxygen saturation, but this technique has not been applied to SCI. It is also unclear whether tissue oxygen tension and saturation monitoring detect the same physiological and pathophysiological changes in CNS injury. Patients with SCI are at increased risk of hemodynamic, respiratory, and systemic deterioration, which can be life threatening and may potentiate secondary injury mechanisms. Several small, uncontrolled studies indicate that invasive monitoring and aggressive treatment of secondary insults in the ICU are associated with decreased mortality, improved neurological recovery, reduced length of hospital stay, and lower complication rates. Strict avoidance and rapid treatment of hypotension and hypoxia are essential in optimizing neurological recovery after SCI. Specialized neurocritical care units provide the ideal environment for the treatment and monitoring of SCI patients. Guideline-based standardized protocols using multimodal monitoring offer a potential novel approach to the management of SCI and may be implemented successfully with the help of new technologies and dedicated treatment facilities.
Management of Spinal Cord Injury in the Intensive Care Unit
Rationale for Spinal Cord Injury Management in the ICU
Hemodynamic Instability
Respiratory System and Complications
Spine Clearance in the Unconscious Patient
Role of the ICU in the Management of Spinal Cord Injury
Multimodal Monitoring for Spinal Cord Injury
Intraspinal Pressure
Spinal Perfusion Pressure and Blood Flow
Conclusion
The core principle of ICU management of SCI is the prevention of secondary injury mechanisms and insults.
It is recommended that patients be treated with vasopressors to keep an SBP > 85 mmHg for a duration of 1 week after injury.
A proactive approach of early intubation under controlled circumstances is recommended to avoid hypoxia and secondary SCI.
CT scanning of the entire cervical spine using thin axial cuts and sagittal and coronal reconstructions is highly sensitive in detecting injuries and is the study of choice for cervical spine clearance in the unconscious patient.
Monitoring in an intensive care setting with early detection and treatment of cardiovascular instability and respiratory failure can potentially reduce complications and improve outcome after SCI.
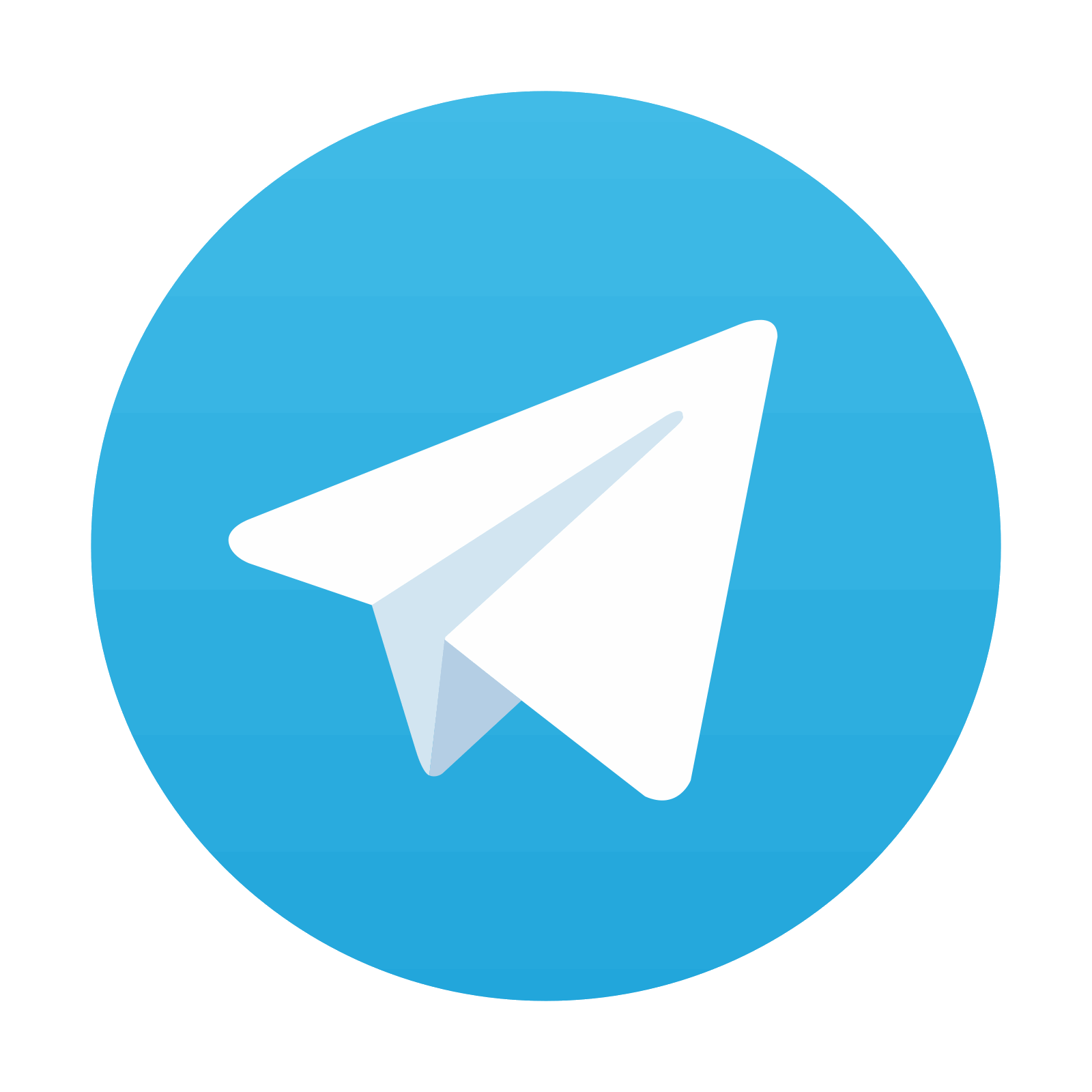
Stay updated, free articles. Join our Telegram channel
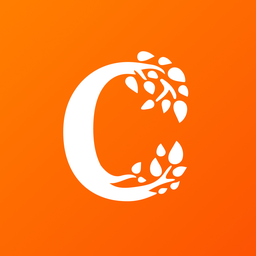
Full access? Get Clinical Tree
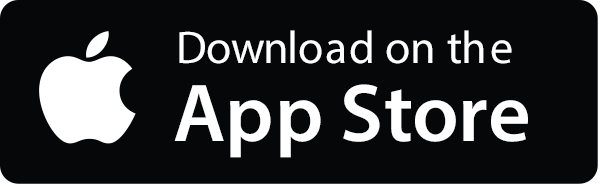
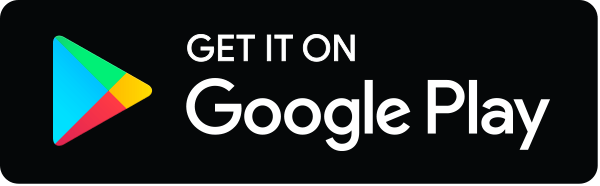