14 INITIAL MANAGEMENT OF TRAUMATIC SHOCK
Rapid recognition of shock is the cornerstone of the early evaluation and treatment of severely injured patients. Sometimes shock is obvious, and even the unsophisticated clinician clearly recognizes the patient in extremis from blood loss. Frequently, however, the findings are substantially more subtle, necessitating increased levels of clinical sophistication and experience to recognize shock, particularly when the victim is able to compensate for blood loss. Shock represents an uncoupling of normal physiologic functioning. Compensatory mechanisms initially strive to maintain homeostasis. This compensation can be robust, particularly in young patients, and patients with substantial blood loss or severe injuries can appear relatively stable initially.1
The importance of early recognition of shock cannot be overemphasized. When untreated or treated very late, shock produces acute organ dysfunction that almost always leads to death, often within 24 hours.2 Late recognition of shock, even if not accompanied by classic signs, produces a profound hypoperfusion insult, with the accumulation of a significant oxygen debt. Patients may survive for the short term but then develop sequential organ failure several days later.2 Despite an increased understanding of injury and resuscitation, we have yet to significantly alter the mortality associated with multiple organ failure.
OXYGEN TRANSPORT
Central to the understanding of the concept of shock is the principle of oxygen transport (Table 14-1). The vast majority of oxygen (98% to 99%) exists in the body bound to hemoglobin. A small portion is freely dissolved in plasma. Thus oxygen content is a function of hemoglobin and oxygen saturation. Cardiac output delivers oxygen to the periphery. The cells then unload the amount of oxygen they need, and the unneeded portion is returned to the heart via the venous circulation. The oxygen extraction ratio is approximately 25%.
The ability of the cell to unload oxygen can be affected by a number of factors. The oxyhemoglobin dissociation curve governs the principles of oxygen affinity to the hemoglobin molecule (Figure 14-1). Some conditions such as alkalosis, hypothermia, or a loss of 2,3-diphosphoglycerate (2,3-DPG) increase oxygen’s affinity for hemoglobin, thus impeding the body’s ability to unload oxygen at the cellular level. Conversely, acidosis, hyperthermia, and increases in 2,3-DPG, shift the curve in the opposite direction, allowing for increased peripheral oxygen unloading. These relationships can be dynamic, particularly in a patient with complicated diagnoses.
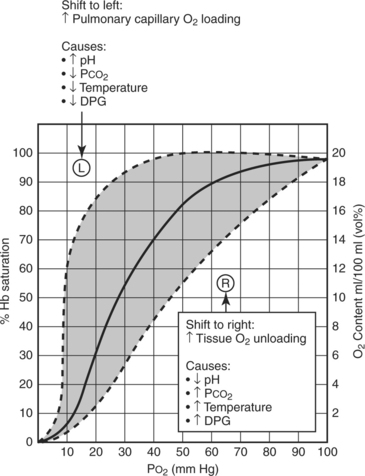
FIGURE 14-1 Oxyhemoglobin dissociation curve.
(From Moms MT: Adult respiratory distress syndrome. In Secour VH, editor: Multiple organ dysfunction & failure, ed 2, St. Louis, 1996, Mosby, 174.)
At some point, oxygen demand can exceed oxygen supply. As compensation fails, the cells must shift to anaerobic metabolism to generate the high-energy phosphate compounds needed for cellular metabolism.3 Anaerobic metabolism is an extremely inefficient means of generating adenosine triphosphate (ATP)—approximately 5% as efficient as aerobic metabolism using the Krebs cycle.4 In addition, lactate is a byproduct of anaerobic metabolism. Lactate may depress cardiac function and limit oxygen delivery. As acidosis worsens, so does cardiac performance, and ultimately death ensues.
Shock can then be defined as the hemodynamic manifestations of any state in which oxygen demand exceeds oxygen supply or utilization regardless of the underlying cause. Thus all shock can be defined as inadequate cardiovascular performance. Given the nature of injury, we attempt to estimate peripheral oxygen delivery through the use of blood pressure or target organ function. Although this practice has the advantage of being quick and readily available in the clinical arena, these parameters are at best nonspecific and in some cases can lead to faulty decision making. Some patients who are hypotensive may not be in shock. Conversely, many patients who are not hypotensive may be in shock. Recognizing this possibility and knowing when it is necessary to obtain more data are key to optimizing care, particularly in patients with complex diagnoses.
PATHOPHYSIOLOGY OF SHOCK
PHYSIOLOGY OF BLOOD LOSS
Blood loss is the most common cause of inadequate cardiovascular performance after injury.5 It can be accompanied by intravascular volume loss from extravasation of plasma into body cavities (e.g., peritoneal cavity) or the interstitium of injured tissues further depressing cardiovascular performance. Fluid losses may also occur through open wounds. Initial attempts to compensate for blood loss are multifactorial. Catecholamine-mediated systemic vasoconstriction is primarily responsible for the increase in systemic vascular resistance seen in many shock states. This allows for the shunting of blood flow away from nonessential organs. Blood is initially directed away from the skin and skeletal muscle, maintaining flow to the more central vascular beds. Ultimately, as blood loss continues, the only core organs protected are the most essential—the heart and brain. Under normal conditions, the perfusion pressure and degree of smooth muscle tone in the supplying vessels determine regional blood flow to any individual organ. As organisms bleed, autoregulatory mechanisms attempt to maintain a steady-state pressure flow to these vital organs. Thus tissue hypoxia will initially be more marked in tissues and organs in which autoregulatory compensation fails.
Tachycardia, a narrowing of pulse pressure, and loss of capillary refill are thought to be the first measurable signs of blood volume loss (Table 14-2). Presentation of these clinically measurable parameters indicates that initial mechanisms thought to provide compensation for inadequate intravascular volume are activated. As blood loss continues, there may be a stepwise change in these and other parameters. When blood loss approximates 40% to 50% of total circulating blood volume (>2 L in a 70-kg man), compensation fails and patients develop bradycardia, obtundation, and cardiac arrest.6 This stepwise change in vital signs allows for estimation of the amount of blood loss and can be used to direct care. For instance, patients who are stable (i.e., normotensive and not tachycardic) could be presumed to have a minor injury and have suffered less than a 15% loss in circulating blood volume. Those who are initially somewhat hypotensive or tachycardic and who respond to initial resuscitation may then be classified as “stabilizable.” Although they must be presumed to have suffered a significant blood loss, they are generally able to undergo diagnostic evaluation to precisely identify the source of blood loss. Some of these patients may even be managed nonoperatively. Patients who present in extremis and do not respond to initial resuscitation must be presumed to have serious ongoing blood loss. Their initial evaluation should be tailored to minimize time, and hemorrhage must be arrested immediately.
Unfortunately, vital signs are often imprecise and may incorrectly classify patients with marginally compensated shock as stable. Tissue oxygen extraction, as measured by either mixed venous or central venous oxygen saturation, is much more specific and correlates reliably with blood loss (Figure 14-2). This has been demonstrated in controlled laboratory models of both anesthetized and unanesthetized canine hemorrhage.7 It has also been investigated in humans who presented to an emergency department with mechanisms of injury suggestive of acute blood loss but who initially seemed to be hemodynamically stable.8 Forty percent of these stable patients had central venous oxygen desaturation, although their blood pressure and pulse rate were similar to those of patients with normal central venous oxygen saturations. Central venous oxygen desaturation was used to reliably predict major blood loss, such as large hemothoraces or hemorrhage from pelvic fractures.
Urine output has been reported to be a reliable indicator of the depth of shock.6 Patients who are oliguric should be presumed to be underresuscitated. Patients who are nonoliguric are generally deemed stable. However, work from the authors’ laboratory seems to refute those conclusions.9 In a porcine model of nonhypotensive shock, animals that lost 15% of their total circulating volume behaved as expected by becoming oliguric. They held on to both salt and water, as evidenced by low urine sodium concentrations. In contrast, animals bled more severely—losing 21%, 27%, and 35% of the total circulating blood volume—all developed an acute salt-wasting nephropathy and acute tubular insufficiency. These animals had high urine-sodium concentrations, and their urine output was not different from that of control animals.
Other factors may alter cardiac performance and perfusion parameters after blood loss. One is mechanism of injury. Patients who have sustained blunt trauma respond differently than those with penetrating injuries. Penetrating trauma approximates a pure blood loss situation. A tissue crush injury that accompanies blunt trauma, however, can stimulate a cascade that, even acutely, liberates vasoactive mediators that can affect hemodynamic performance. Patients with this type of injury may develop a high cardiac output/low vascular resistance state similar to sepsis. Ethanol use, which is commonly associated with trauma, has been shown to modulate plasma norepinephrine concentrations after injury, blunting the vascular response and moderating the effect of adrenergic stimuli.10 Street drugs such as cocaine may also affect cardiovascular performance. Animals pretreated with cocaine and then subjected to blood loss had a blunted response to hemorrhage compared with control animals.11
Some vascular beds may respond differently than others. Global parameters such as mixed venous oxygen saturation reflect a mixing of blood from all vascular beds. Identification of the most responsive vascular beds could allow earlier diagnosis of blood loss. The mesenteric circulation is exquisitely sensitive to falls in preload.12 Blood flow to the gut is reduced primarily via vasoconstriction caused by elevated renin angiotensin activity. This is opposed to the renal circulation, in which hemorrhagic hypotension may activate renal vasodilatory signals via local release of prostaglandins and nitric oxide. Monitoring of mesenteric circulation could potentially provide early warning signals to critical but nonhemodynamically significant hemorrhage. The adequacy of flow can be measured via intracellular pH. Indirect measurement of this parameter via gastric tonometry catheters has been demonstrated to predict adequacy of resuscitation in the general class of intensive care unit (ICU) patients.13 Unpublished work from the authors’ laboratory has demonstrated that gastric tonometry is an extremely sensitive indicator of blood loss that changes before other vital signs. In addition, the authors have demonstrated that reduction in gastrointestinal oxygen delivery is accompanied by oxygen supply dependency.
NEUROGENIC SHOCK
Efferent sympathetic neurons in the thoracic and cervical spinal cord are dependent on descending input from the hypothalamus and brainstem for vasomotor control. Brainstem damage or spinal cord injury at or above the T6 level may then produce neurogenic shock. In this type of shock, loss of vasoconstrictor-sympathetic activity leads to vascular collapse. With loss of vasomotor control, even previously adequate blood volume becomes incapable of filling the now dilated capacitance vessels. Bradycardia and hypotension are common. However, unlike hemorrhagic shock, neurogenic shock is characterized by a slow pulse rate and warm, dry skin. This is a relative hypovolemia resulting from decreases in peripheral vascular and cardiac sympathetic activity. Hemodynamic patterns can be variable. Decreases in systemic vascular resistance, central venous pressure, and pulmonary capillary wedge pressure are nearly universal. Cardiac output, on the other hand, may be low, normal, or elevated. The loss of vascular resistance allows unimpeded forward flow from the heart. These salutary effects on cardiac performance may be counterbalanced, however, by the negative effect of inadequate preload from the vasodilation. Refer to Chapter 23 for information on management of neurogenic shock.
The hemodynamics of neurogenic shock may be further complicated by differences in mechanism of injury. A spinal cord injury may be accompanied by blood loss, which can exacerbate any of the hemodynamic consequences. The lack of sympathetic response may also mask the signs of acute blood loss. Soderstrom et al demonstrated that nearly 70% of patients with cervical spine injury and blunt trauma are hypotensive from their spinal cord injury, not concomitant blood loss.14 In contradistinction, a series of patients with penetrating spinal cord injuries found that neurogenic shock explained hypotension in approximately 20% of patients.15 This underscores the need to completely evaluate all patients regardless of the obvious nature of one particular injury.
CARDIOGENIC SHOCK
The most frequent cause of cardiogenic shock is acute myocardial infarction.5 This can accompany injury, particularly in elderly patients. Cardiogenic shock occurs when 35% to 40% of the left ventricular muscle mass is damaged.16 The resulting impairment in left ventricular function produces elevated end-systolic volumes and filling pressures. This in turn limits diastolic filling. Cardiac output falls and produces often profound hypotension. Coronary perfusion is thereby decreased, potentially extending the area of ischemia. This ischemia further drops cardiac output and creates an ever-widening cyclic process, which will result in patient death unless truncated early.
Patients with acute cardiac failure after injury are a special subset of patients and present many challenges. Peripheral oxygen delivery needs must be met to maintain aerobic metabolism. Inotropic support may be necessary to support the failing heart. Cardiac protection with beta blockade, afterload reduction, and conservative fluid administration will help preserve myocardium but will limit forward flow. Despite all interventions, cardiogenic shock in this setting is often a lethal state.17
SEPTIC SHOCK
Pure septic shock resulting from infection is unusual immediately after acute trauma. The only exception may occur in patients with grossly contaminated wounds. Significant soft tissue infection is seen in patients with multiple long-bone fractures, and these patients occasionally present with septic shock several hours after injury. The mediator release stimulated by significant soft tissue injury, especially when accompanied by gross contamination or dead muscle, often presents in a manner similar to septic shock.
INJURY DEMOGRAPHICS
Last year nearly 40,000,000 people were seen in our nation’s emergency departments as a result of unintentional injury. More than 2 million of them required hospitalization. In 2004 the number of deaths from unintentional injury increased for the seventh consecutive year, to 167,184.18 Experts expect this trend to continue.
Patients die in a trimodal distribution after injury. The vast majority of deaths occur at the scene from immediately life-threatening airway compromise, brain injury, and/or exsanguinating hemorrhage. In fact, there is little that trauma care can do to improve these patients’ survival other than implement trauma prevention strategies. An additional 20% of trauma deaths occur in the early phase, generally from exsanguinating hemorrhage.19 It is this group of patients in whom prehospital and emergency department decision making can potentially have an effect. The last group of patients die later from multiple organ failure. The cause of multiple organ dysfunction and subsequent failure usually originates from a systemic inflammatory response initiated by injury or sepsis. This group represents approximately 10% of trauma deaths.19 Some of these deaths may be prevented by limiting shock and using optimal resuscitation strategies. The exact percentage of lives that can be saved by altering trauma care, although not insignificant, remains unknown.
Blunt Trauma
Blunt trauma is the most common mechanism of injury in the United States.20 The mechanics of blunt injury involve a compression or crushing mechanism via energy transmission and direct injury. If compressive, shearing, or stretching forces exceed the tolerance limit of a tissue or an organ, the tissues are disrupted. This may result in injury to the solid viscera, such as the liver or spleen, or rupture of the hollow viscera, such as the gastrointestinal tract.
The most common cause of death and disability from unintentional injuries worldwide is the motor vehicle crash. In 2003 the number of deaths from motor vehicle-related incidences in the United States alone rose to more than 43,000, and nearly 21,000,000 people suffered some disabling injury.18 The injury patterns seen with motor vehicle crashes are difficult to predict because a number of factors contribute to them. Certainly the speed of the vehicle and the surface that it strikes are important in determining the vectors of energy. The use of restraint systems such as seat belts and air bags also influences both the severity of injury and the type of injury seen. A high-speed, head-on collision into a bridge abutment with an unrestrained driver at the wheel is far different from the car traveling at 20 mph that strikes a tree with a glancing blow with a driver protected by a seat belt and an air bag. Both are motor vehicle crashes, but the injury patterns will be substantially different.
Falls are the second leading cause of unintentional death in the United States and were responsible for more than 7,500,000 emergency department visits in 2002. They continue to account for more than 20% of all injury-related hospital admissions.18 Falls from a height can produce a unique pattern of injury. The degree of injury is a function of the distance of the fall, the surface on which the victim lands, and whether the fall is broken by objects during flight. The center of gravity in adults resides in the pelvis, whereas in young children the center of gravity is in the head. Therefore children who fall a great distance often land on their head and thus are likely to suffer severe traumatic brain injury. Adults, on the other hand, often right themselves as they fall and land on their feet. The force is then transmitted up the axial skeleton into the spine. Lower extremity, pelvic, and thoracolumbar spinal fractures are common, as well as injuries to the retroperitoneal viscera. Brain injury and intraabdominal injury, however, are relatively rare.
DIAGNOSIS AND TREATMENT OF SHOCK
PREHOSPITAL CARE
The goal in caring for any trauma patient is to identify those most severely injured as early as possible and then to minimize the time from injury to definitive therapy. Unfortunately, diagnosis of shock in the field can be difficult. Blood pressure and pulse rate are crude measures of the adequacy of peripheral perfusion,7 yet little else is available to the prehospital care provider. These individuals must make rapid decisions based on a paucity of information. Thus in most emergency medical services (EMS) systems the tendency has been to overtriage as opposed to undertriage to avoid delivering a patient to an institution not capable of providing adequate care.
Several philosophies exist regarding the care of patients in the prehospital setting who are confirmed or suspected to be in shock (Table 14-3). The first is to attempt to stabilize these patients in the field. This “stay and play” philosophy is based on an assumption that time in the field can be well spent stabilizing the patient’s physiologic status.21 Correction of oxygenation or ventilatory problems can be lifesaving. The insertion of intravenous (IV) catheters for fluid resuscitation can help correct cardiovascular instability. A brief neurologic assessment can identify patients at high risk for severe brain injury, particularly those with impending herniation. Therapy can then be directed and triage possibly improved. Unfortunately, the time involved in even rapid evaluation and temporary stabilization often causes concern.
TABLE 14-3 Field Resuscitation Strategies
Stay and Play | Load and Go | |
---|---|---|
Time at Scene | Longer (20-30 min) | Shorter (<10 min) |
Interventions | ||
Appropriate locale | Rural | Urban |
Advantages | * Shorter time to definitive care | |
Disadvantages |
Modified from Border JR, Lewis FR, Aprahamian C et al: Panel: prehospital trauma care—stabilize or scoop and run, J Trauma 23:708-711, 1983; and Gold CR: Prehospital advanced life support vs “scoop and run” in trauma management, Ann Emerg Med 16:797-801, 1987.
Excessive time in the field can prolong the interval from injury to definitive therapy. Proponents of the “scoop and run” philosophy believe that only immediately life-threatening problems, such as airway issues, should be addressed in the field.22 Patients should be immobilized on spine boards and external hemorrhage controlled with direct pressure. All other therapies should be attempted only en route. Prolonging field time even long enough to insert an IV catheter may negatively alter ultimate outcome.
In 1983 Smith et al reviewed the prehospital care of 52 trauma victims who underwent attempts at in-field stabilization.23 In all cases the IV insertion time was longer than the transport time. Failure of line placement occurred 28% of the time and occurred most often in those patients most severely injured. The average IV insertion time in the field was between 10 and 12 minutes. Border et al estimated that blood loss during this time may be in excess of 1500 ml in severely injured patients.24
This issue was further investigated by clinicians at Los Angeles County Hospital.25 In this study nearly 6000 trauma patients admitted via the emergency department were evaluated for field time and mode of transportation. Patients who were transported by private vehicle as opposed to the city EMS system got to the hospital statistically significantly faster. The main reason for this appeared to be the amount of time the prehospital personnel spent in the field attempting to stabilize the patient. The moderate to severely injured patients (Injury Severity Score [ISS] 15-25) arriving at the hospital without the benefit of city ambulance transport had a lower mortality than their EMS-transported counterparts. These compelling data argue strongly in favor of “scoop and run.”
Fluid administration in the prehospital arena has evolved considerably in the past several years. Original treatment algorithms called for establishment of large-bore IV access and instillation of 2 L of crystalloid fluid as rapidly as possible, with the goal of normalizing both blood pressure and pulse rate. Early in the twentieth century, however, Cannon et al suggested that IV fluids administered in the field to patients with hypotension could be injurious.26 He theorized that crystalloid fluid would serve only to displace hemostatic clots that may have formed on injured blood vessels as blood pressure fell. In addition, he felt that if bleeding persisted despite hypotension, administration of IV fluids would not positively influence outcome.
This thought was corroborated scientifically in 1965, when Shaftan et al investigated this theory in a canine hemorrhagic shock model.27 They investigated various resuscitation regimens to elucidate the relative effects of pressure and volume on the hemodynamic response to crystalloid fluid resuscitation. They demonstrated that the time to hemostasis was longest and blood loss greatest in animals resuscitated to normal volume status and blood pressure. Subsequently, numerous animal models, most often a porcine aortotomy model of hemorrhagic shock, have been used to verify these findings.28–30 All these investigations demonstrated that intraperitoneal blood loss is greatest when animals are resuscitated to normal blood pressure. The excessive amount of crystalloid fluid required to maintain normal blood pressure seems to hemodilute the available hemoglobin and increase intraperitoneal blood loss, presumably by displacing the clot that forms on top of the injured aorta at the time of hypotension. In 1994 Bickell et al randomized patients with penetrating torso trauma and hypotension in the field to receive no fluid or standard crystalloid fluid, with a target systolic blood pressure of 100 mm Hg.31 Patients in the fluid-restricted group received fluids only at the time of anesthetic induction for surgical control of their hemorrhagic shock. In this study there was a statistically significant survival advantage to fluid restriction.
Thus it seems that moderate hypotension, although potentially detrimental, is in fact relatively well tolerated, at least for short periods. This may be better than the alternative of increased blood loss and hemodilution with crystalloid resuscitation to attain normal blood pressure. In patients with traumatic brain injury, hypotension has been shown to double mortality.32 Thus, fluid restriction is probably not wise in these patients. Alternatively, geriatric patients with limited cardiovascular reserve may require different treatment algorithms to avoid development of acute cardiac ischemia, which almost certainly will lead to death.
The type of fluid used in the prehospital environment has likewise been called into question (Table 14-4). In most EMS systems, crystalloid fluid is the preferred volume expander. There is, however, some evidence that hypertonic saline may have the advantage of more rapid restoration of cardiovascular function with a smaller volume of fluid. As little as 4 ml/kg, if given rapidly, may have the same hemodynamic effect as several liters of crystalloid.33 The increase in cardiac output and mean arterial pressure with hypertonic saline results from an osmotic shift of fluid from the interstitial and intracellular spaces into the intravascular space. This is mediated by rapid increases in serum osmolarity that accompany the infusion of 7.5% sodium chloride, which has a milliosmolarity of 2565. The addition of dextran prolongs this plasma expansion.34 In several animal models of hemorrhage, hypertonic saline/dextran improved stroke volume and decreased both heart rate and systemic vascular resistance.35,36 Enthusiasm for use of hypertonic solutions has been counterbalanced by several small studies suggesting that rebleeding and hemodynamic instability may occur after the infusion of hypertonic saline when blood loss is not fully controlled.37,38 Hypertonic saline and hypertonic saline/dextran have been investigated in several randomized prospective trials in humans. Mattox et al demonstrated increases in blood pressure in a multi-institutional study on the use of hypertonic saline in the prehospital arena.39 In the subset of patients requiring emergency life-saving surgery to control hemorrhage, survival was statistically significantly better in those who were treated with hypertonic saline compared with patients treated with dextran or crystalloid fluid. Vassar et al investigated this issue in four separate randomized trials during the early 1990s.40–42 They were unable to demonstrate any survival advantage in the broad group of patients randomized to hypertonic saline compared with crystalloid fluid. However, in the subset of patients with severe traumatic brain injury, there appeared to be a survival advantage to using hyperosmotic resuscitation. This may have been due to a decrease in secondary brain injury from the more rapid restoration of cardiovascular function or decreases in intracranial pressure, as seen with the administration of hypertonic saline. The cerebral osmotic effects of hypertonic saline are similar to those of mannitol. In a meta-analysis Wade et al demonstrated a distinct survival advantage for patients with serious traumatic brain injury treated with hypertonic saline.34 In a more recent study, 229 hypotensive patients with severe traumatic brain injury were randomized to receive a rapid IV infusion of either 250 ml of Ringer’s lactate solution or 250 ml of 7.5% saline in addition to other resuscitation fluid provided in the prehospital setting, Cooper et al found a small trend toward greater survival in those patients who received hypertonic saline during resuscitation. However, neurologic outcome at 6 months after injury was identical to that found in the group that received only crystalloid resuscitation.43
In the late 1980s, military antishock trousers (MAST) were thought to be useful in the resuscitation scheme after trauma. MAST were thought to autotransfuse volume by applying pressure to the capacitance vessels in the lower extremities. Added advantages included the potential stabilization of long-bone fractures and reduction of pelvic fractures. Data, however, have failed to demonstrate any real utility to MAST. In fact, increases in blood pressure seen with the use of MAST seem to be secondary to increases in systemic vascular resistance, and MAST do not augment cardiac preload. In a randomized trial of 911 patients, Mattox et al demonstrated no survival advantage with use of MAST.44 Wangensteen et al reported that pneumatic external compression without concomitant intravascular volume replacement accelerated the development of lactic acidosis and decreased the survival rate in animal models.45 In general, the widespread use of MAST has fallen out of favor, and many EMS systems have abandoned their use in the prehospital arena. However, there may be some uses for MAST. They may be useful during long transports to help support at least some blood pressure when no other mode of therapy is available. MAST can be helpful as a temporary splint in patients with extensive long-bone fractures. In addition, they can be helpful in patients with complex pelvic fractures by reducing the fracture, limiting pelvic volume, and potentially reducing blood loss. They do, however, introduce the possibility of cardiovascular effects resulting from increased intraabdominal pressure. Potential complications of MAST have prompted many trained prehospital and emergency room practitioners to use a sheet or binder wrapped around the circumference of the pelvis to achieve the same temporary stabilization without the MAST-related adverse effects (e.g., increased intrathoracic, intraabdominal pressures).
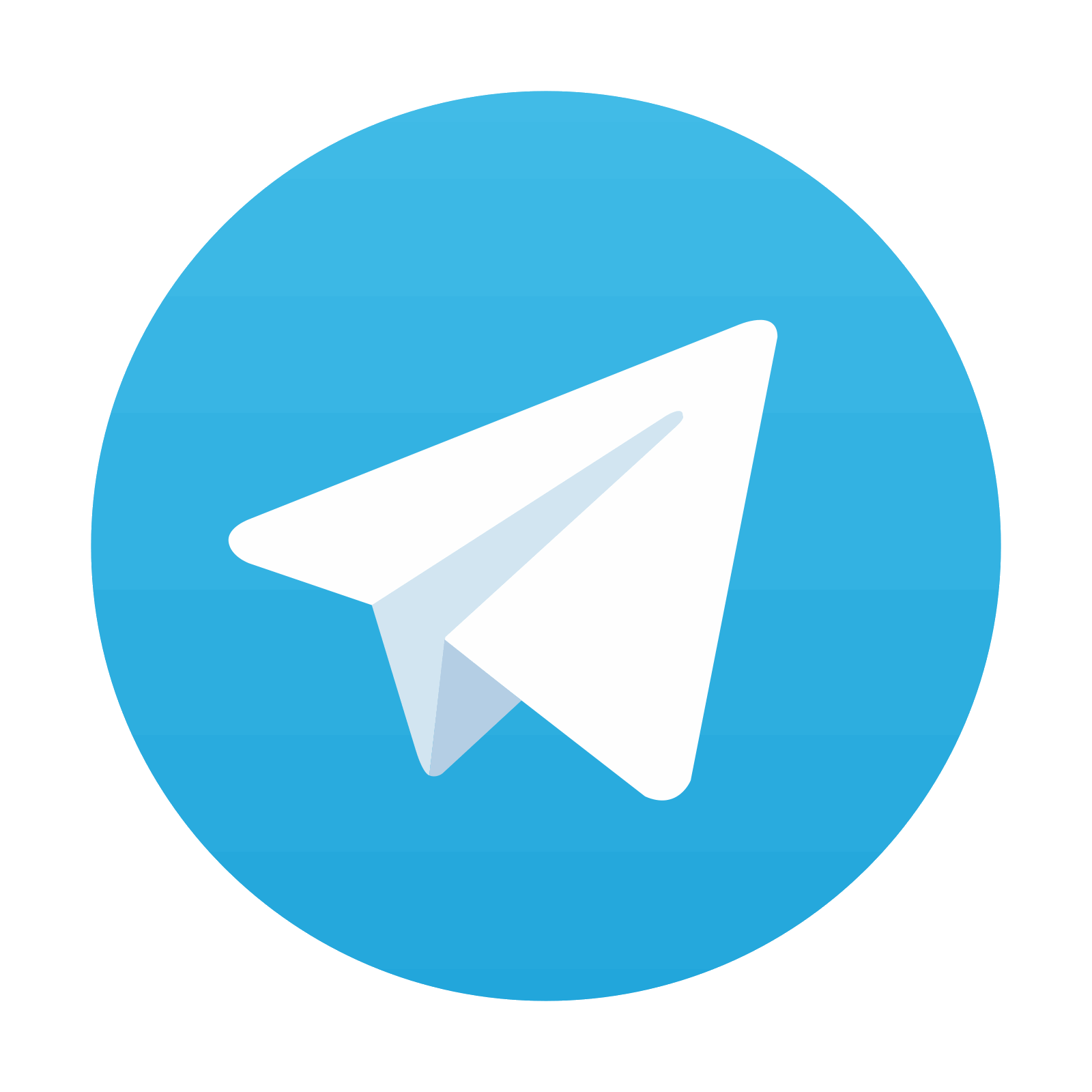
Stay updated, free articles. Join our Telegram channel
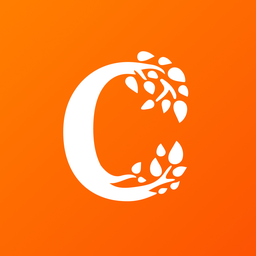
Full access? Get Clinical Tree
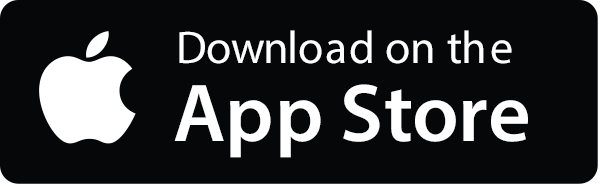
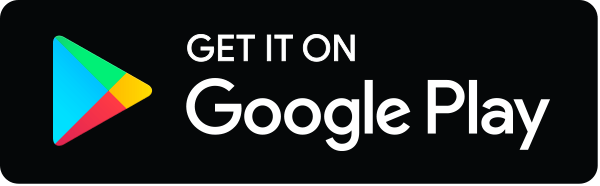