CHAPTER 6 | Gait and Posture Analysis |
OVERVIEW
The assessment of symmetry within locomotion and posture is critical in the evaluation of neuromusculoskeletal dysfunction. For most individuals, gait or posture is an innate characteristic, as much a part of their personality as their smile. Indeed, many people can be recognized in a group by their gait or posture. The purpose of this chapter is to describe the various components of gait and posture and to provide the clinician with the necessary tools for the analysis of each.
Gait and the Gait Cycle
The lower kinetic chain has two main functions: to provide a stable base of support (BOS) in standing, and to propel the body through space with gait. During gait, the body follows the least restrictive pathway in the most efficient manner.1 In order to maintain this efficiency, the body attempts to maintain a level center of gravity (COG) in all planes.1 While maintaining a static equilibrium of forces, the objective with mobility is to create and control dynamic, unbalanced forces to produce movement.2 Gait is thus an example of controlled instability. It is not clear whether gait is learned or is preprogrammed at the spinal cord level. However, once mastered, gait allows us to move around our environment in an efficient manner, requiring little in the way of conscious thought, at least in familiar surroundings. The evolution of bipedal gait has allowed the arms and hands to be free for exploration of the environment. Even though gait appears to be a simple process, it is prone to breakdown. Despite individual gait patterns being characterized by significant variation, only three essential requirements are necessary for efficient locomotion: progression, postural control, and adaptation:3
Progression. The fall that occurs at the initiation of gait so that an individual must take the first step is controlled by the central nervous system (CNS).4 The CNS computes in advance the required size and direction of this fall toward the supporting foot. Progression of the head, arms, and the trunk is initiated and terminated in the brainstem that maintains a central pattern generator (CPG) through the spinal cord (see Chapter 3). The locomotor CPG produces self-sustaining patterns of stereotype motor output resulting in gait-like movements. In addition, gait relies on the control of the limb movements by reflexes. Two such reflexes include the stretch reflex and the extensor thrust. The stretch reflex is involved in the extremes of joint motion, whereas the extensor thrust may facilitate the extensor muscles of the lower extremity during weight bearing.5 Both the CPG and the reflexes that mediate afferent input to the spinal cord are under the control of the brainstem and are therefore subconscious.6 This would tend to indicate that verbal coaching (i.e., feedback that is processed in the cortex) regarding an aberrant gait pattern might be less effective than a sensory input that will elicit a brainstem-mediated postural response.2
Postural control. Postural control is dynamically maintained to appropriately position the body for efficient gait.
Adaptation. Although central pattern generation occurs independent of any sensory input, afferent information from the periphery can influence the CPG. Adaptation is achieved by adjusting the central pattern generated to meet task demands and environmental demands.
Gait, therefore, is initiated grossly in the spinal cord and then fine-tuned by the higher brain centers.2 In patients who have developed dysfunctional gait patterns, physical therapy can help to restore this exquisite evolutionary gift.7 Pain, weakness, and disease can all cause a disturbance in the normal rhythm of gait. However, except in obvious cases, abnormal gait does not always equate with impairment.
In addition to neural input, normal human gait also involves a complex synchronization of the cardiopulmonary and muscular systems in which the cardiopulmonary system produces the energy required for gait. Normal gait requires concentric, eccentric, and isometric contractions of the foot, ankle, knee, hip, and trunk as well as training to maintain an upright posture.1
Walking involves the alternating action of the two lower extremities. This walking pattern is studied as a gait cycle. A gait cycle is defined as the interval of time between any of the repetitive events of walking. Such an event could include the point when the foot first contacts the ground to the point when the same foot contacts the ground again. The seven intervals that occur during the gait cycle include:
- Loading response
- Midstance
- Terminal stance
- Preswing
- Initial swing
- Midswing
- Terminal swing
These intervals occur during two phases, the stance phase and the swing phase (Fig. 6-1). Four of the intervals occur in the stance phase, and the remaining three occur during the swing phase (Fig. 6-2).
FIGURE 6-1 The two phases of gait.
FIGURE 6-2 The intervals of gait.
- Stance. The stance phase (Fig. 6-1) takes about 0.6 seconds during an average walking speed. This phase constitutes approximately 60% of the gait cycle (Fig. 6-1) and describes the entire time the foot is in contact with the ground and the limb is bearing weight. The stance phase begins when the foot makes contact with the ground (initial contact) and concludes when the ipsilateral foot leaves the ground (toe-off) (Fig. 6-2). The initial contact interval accounts for the first 10% of the gait cycle and describes the phase when one foot is coming off the floor while the other foot is accepting body weight and absorbing the shock of initial contact (Fig. 6-2). Because both feet are in contact with the floor, this phase is referred to as double support (Fig. 6-1). The phase of double support occurs twice during a single gait cycle and accounts for approximately 20% of the gait cycle (Fig. 6-1). The four intervals which occur in this phase include the loading response, midstance, terminal stance, and preswing (see Fig. 6-2).
- Loading response. The loading response interval begins as one limb bears weight while the other leg begins to go through its swing phase. The extent to which eccentric muscle tension counteracts the effect of gravity on the body mass during the loading response to ground contact determines both the velocity and amplitude of motion that occurs at any given joint in the lower extremity.8
- The midstance interval, representing the first half of the single-limb support task (Fig. 6-2), begins as one foot is lifted and continues until the body weight is aligned over the forefoot.
- The terminal stance interval (Fig. 6-2) is the second half of the single-limb support task. It begins when the heel of the weight-bearing foot lifts off the ground and continues until the contralateral foot strikes the ground.
- The preswing interval (Fig. 6-2) begins with the initial contact of the contralateral limb and ends with the ipsilateral toe-off. During this phase, the stance leg is unloading the body weight to the contralateral limb and preparing the leg for the swing phase.
- The midstance interval, representing the first half of the single-limb support task (Fig. 6-2), begins as one foot is lifted and continues until the body weight is aligned over the forefoot.
- Loading response. The loading response interval begins as one limb bears weight while the other leg begins to go through its swing phase. The extent to which eccentric muscle tension counteracts the effect of gravity on the body mass during the loading response to ground contact determines both the velocity and amplitude of motion that occurs at any given joint in the lower extremity.8
- Swing. Gravity and momentum are the primary sources of motion for the swing phase.5 The swing phase constitutes approximately 40% of the gait cycle (Fig. 6-2) and describes the phase when the foot is not in contact with the ground. The swing phase begins as the foot is lifted from the ground and ends when the ipsilateral foot makes contact with the ground again. The three intervals which occur in this phase include initial swing, midswing, and terminal swing:
- Initial swing (Fig. 6-2). This interval begins with the lifting of the foot from the floor and ends when the swinging foot is opposite the stance foot. It represents the 60–73% phase of the gait cycle (Fig. 6-2).
- Midswing (Fig. 6-2). This interval begins as the swinging limb is opposite the stance limb and ends when the swinging limb is forward, and the tibia is vertical. It represents the 73–87% phase of the gait cycle (Fig. 6-2).
- Terminal swing (Fig. 6-2). This interval begins with a vertical tibia of the swing leg with respect to the floor and ends the moment the foot strikes the floor. It represents the last 87–100% of the gait cycle (Fig. 6-2).
- Initial swing (Fig. 6-2). This interval begins with the lifting of the foot from the floor and ends when the swinging foot is opposite the stance foot. It represents the 60–73% phase of the gait cycle (Fig. 6-2).
The precise duration of the gait cycle intervals depends on a number of factors including, but not limited to, age, degree of impairment, attention to another task, health status, motor control, muscle performance, endurance and habitual activity levels, walking environment, and the patient’s walking velocity.9 Walking velocity is defined as the distance a body moves in a given time and is thus calculated by dividing the distance traveled by the time taken. Normal free gait velocity on a smooth and level surface averages about 1.2–1.4 m/s for adults, with speed varying by gender, age and anthropometrics, with men being about 5% faster than women.10
Running involves both a stance phase (30%) and a swing phase (70%), with increased speed showing an increase in the swing to stance ratio. Running also involves a float phase, during which there is no foot contact with the ground, and potential energy is greatest, and which comprises 30% of the running cycle.11
GAIT PARAMETERS
A normal gait pattern is a factor of a number of parameters.
Step Width
The step width is the distance between both feet. The normal step width, which is considered to be between 5 and 10 cm (2–4 in), forms the BOS during gait. The size of the BOS and its relation to the COG are important factors in the maintenance of balance (see Chapter 1) and, thus, the stability of an object. As the COG moves forward with each step, it briefly passes beyond the anterior margin of the BOS, resulting in a temporary loss of balance.13 This temporary loss of equilibrium is counteracted by the advancing foot at initial contact, which establishes a new BOS. A larger than normal step width or BOS is observed in individuals who have muscle imbalances of the lower limbs and trunk, as well as those who have problems with overall static and dynamic balance.14 Assistive devices, such as crutches or walkers, can be prescribed to increase the BOS and, therefore, enhance stability. The step width should be seen to decrease to around zero with increased speed. If the step width decreases to a point below zero, crossover occurs, whereby one foot lands where the other should, and vice versa.
Step Length
Step length is measured as the distance between the point of initial contact of one foot and the point of initial contact of the opposite foot. The average step length is about 72 cm (28 in). The measurement should be equal for both legs.
Stride Length
Stride length is the distance between successive points of foot-to-floor contact of the same foot. A stride is one full lower extremity cycle. Two step lengths added together make the stride length. The average stride length for normal adults is 144 m (56 in).10
Typically, the step and stride lengths do not vary more than a few centimeters between tall and short individuals. Men typically have longer step and stride lengths than women. Step and stride lengths decrease with age, pain, disease, fatigue, and as the speed of gait decreases.16,17 A decrease in step or stride length may also result from a forward head posture, a stiff hip, or a decrease in the availability of motion at the lumbar spine. The decrease in step and stride length that occurs with aging is thought to be the result of a number of factors including an overall decrease in joint range of motion (ROM), and the increased likelihood of falling during the swing phase of ambulation, caused by diminished control of the hip musculature.16,17 This lack of control prevents the aged person from being able to intermittently lose and recover the same amount of balance that the younger adult can lose and recover.
Cadence
Cadence is defined as the number of separate steps taken in a certain time. Normal cadence is between 90 and 120 steps per minute.10 The cadence of women is usually six to nine steps per minute slower than that of men.13 Cadence is also affected by age, decreasing from the age of 4 to the age of 7 years, and then again in advancing years.18
Velocity
The primary determinants of gait velocity are the repetition rate (cadence), physical conditioning, and the length of the person’s stride.10
TABLE 6-1 | Gait Parameters |
Cadence (steps/min) = velocity (m/s) × 120/stride length (m) Stride length (m) = velocity (m/s) × 120/cadence (steps/min) Velocity (m/s) = cadence (steps/min) × stride length (m)/120 |
Data from Levine D, Whittle M. Gait Analysis: The Lower Extremities. La Crosse, WI: Orthopaedic Section, APTA, Inc.; 1992. |
As gait speed increases, it develops into jogging at approximately 2.0–2.7 m/s,29 and then running, with changes in each of the intervals. For example, as speed increases, the stance phase decreases and the terminal double stance interval disappears altogether, so that there are alternating single extremity support intervals that are separated by a period of no ground contact by either extremity. This produces a double unsupported interval.30 Initial contact with the ground is normally made by the heel when walking, which is also the case for more than 75% of individuals while running.31 Normal running gait begins with lateral heel strike, followed by foot pronation during midstance, and foot supination during pushoff. The rear foot strike running pattern is facilitated by the elevated and cushioned heels of modern running shoes during which ground reaction forces (GRFs) (see next section) reach 1.5–3 times body weight.32 A current trend in rehabilitation has centered on modifying running technique to prevent, treat, and reduce running injuries. Different running styles are defined by the portion of the foot segment the makes initial contact with the ground. These patterns include rear foot strike, midfoot strike, and forefoot strike.33 Runners tend to use a heel strike or midfoot strike, whereas sprinters typically use a forefoot strike. Barefoot, or evolution, running, with its emphasis on forefoot striking, has increased in popularity due to its claim that the style produces a significant reduction in GRFs, stride length, and ground contact time. As with walking, running economy can be affected by physiological and biomechanical factors. While forefoot strikers rely more heavily on musculature to aid in cushioning during stance, traditional runners demonstrate a heel strike pattern, secondary to a heavy reliance on footwear and skeletal structures to absorb GRFs.34 While it has been demonstrated that barefoot forefoot strike runners generate smaller impact forces than shorter rear foot strikers, there is no evidence to date that either confirms or refutes improved performance and reduced injuries in barefoot runners, although there is some evidence that the former improves running efficiency.34
Although the motion occurring at each of the lower extremity joints is similar for walking and for running, the required ROM increases with the speed of the activity. As the speed of walking or running increases, so do the forces that act on the various joints, and the joints themselves have to produce greater excursions with greater muscle activity.
Humans have the capacity to run at a broad spectrum of speeds.35 Elite athletes have the ability to achieve maximal running speeds greater than 10 m/s (36 km/h).36 Gait speed can be increased by pushing on the ground more forcefully, pushing on the ground more frequently, or a combination of the two. When speed is initially increased, the priority appears to be pushing on the ground more forcefully as this results in a longer stride length because the body spends more time in the air.37 The lower limb muscles that are responsible for pushing on the ground forcefully during running are the major ankle plantarflexors (soleus and gastrocnemius). At speeds beyond approximately 7.0 m/s, the dominant strategy shifts toward the goal of increasing stride frequency and pushing on the ground more frequently, which results in the force generating capacity of these muscles becoming less effective.35 Instead, as more power at the hip joint is required, the proximal lower limb muscles such as the iliopsoas, gluteus maximus, rectus femoris, and hamstrings become dominant.35
Due to its repetitive nature, running has the potential to create lower extremity pathology. For example, at the hip, they may be microinstability due to repeated hip extension and stretching of the anterior capsule.38
Ground Reaction Forces
An analysis of GRFs is important to quantify the magnitude of forces sustained by body structures during movements and exercise. Newton’s third law states that for every action there is an equal and opposite reaction. GRFs can be influenced by several factors, such as the environment in which the movement is executed (e.g., on land or in water), movement speed, and the weight of the individual performing the exercise. The center of pressure (COP) is the location of the vertical projection of the GRF, and is equal and opposite to the weighted average of all the downward forces acting on the area of contact with the ground.39 The COP is considered to be a reflection of the body’s neuromuscular responses to imbalances of the COG. During gait, vertical GRFs are created by a combination of gravity, body weight, and the firmness of the ground. For example, during running, joint reactive forces through the hip have been demonstrated at five times body weight during single-limb support. Even when immersed in water, stationary running still involves contact forces (see Chapter 8). Under normal conditions, we are mostly unaware of these forces. However, in the presence of joint inflammation or tissue injury, the significance of these forces becomes apparent. The GRF during gait begins with an impact peak of less than body weight and then exceeds body weight at the end of the initial contact interval, dropping during midstance and rising again to exceed the body weight, reaching its highest peak during the terminal stance interval. Thus, there are two peaks of GRF during the gait cycle: the first at maximum limb loading during the loading response and the second during terminal stance.
The GRF vector changes from quite far anterior to the hip joint at initial contact to a progressively posterior position at late stance, when the GRF is posterior to the hip.10,40,41 Peak flexion torque occurs at initial contact but gradually declines, changing to an extension torque in midstance. The extension torque remains until terminal stance.10,40,41 In the frontal plane, a hip abduction moment is generated to counteract the relative hip adduction angle at initial contact.
During the gait cycle, the tibiofemoral joint reaction force has two peaks: the first immediately following initial contact (two to three times body weight) and the second during preswing (three to four times body weight).42 Tibiofemoral joint reaction forces increase to five to six times body weight for running and stair climbing, and eight times body weight with downhill walking.42,43
It is well established that joint angles and GRF components increase with walking speed.44 This is not surprising, because the dynamic force components must increase as the body is subjected to increasing deceleration and acceleration forces when walking speed increases.
Mediolateral Shear Forces
Mediolateral shear in walking gait begins with an initial medial shear (occasionally lateral) force after initial contact, followed by lateral shear for the remainder of the stance phase.10 At the end of the stance phase, the shear shifts to a medial direction because of propulsion forces.
Anteroposterior Shear Forces
Anteroposterior shear forces in gait begin with an anterior shear force at initial contact and the loading response intervals, and a posterior shear at the end of the terminal stance interval.
CHARACTERISTICS OF NORMAL GAIT
Much has been written about the criteria for normal and abnormal gait.10,20,28,40,46–52 Although the presence of symmetry in gait appears to be important, asymmetry in itself does not guarantee impairment. It must be remembered that the definition of what constitutes the so-called normal gait is elusive. Unlike posture, which is a static event, gait is dynamic and as such is protean.
Gait involves the displacement of body weight in a desired direction, utilizing a coordinated effort between the joints of the trunk and extremities and the muscles that control or produce these motions. Any interference that alters this relationship may result in a deviation or disturbance of the normal gait pattern. This, in turn, may result in increased energy expenditure or functional impairment.
Perry and Burnfield10 list four priorities of normal gait:
- Stability of the weight-bearing foot throughout the stance phase.
- Clearance of the NWB foot during the swing phase.
- Appropriate prepositioning (during terminal swing) of the foot for the next gait cycle.
- Adequate step length.
Gage et al.18 added a fifth priority, energy conservation. The typical energy expended in normal gait (2.5 kcal/min) is less than twice that spent while sitting or standing (1.5 kcal/min).18 Two-dimensional kinetic data have revealed that approximately 85% of the energy for normal walking comes from the plantarflexors of the ankle, and 15% from the flexors of the hip.54 It has been proposed that the type of gait selected is based on metabolic energy considerations.55 Current commonly used parameters to measure walking efficiency include oxygen consumption, heart rate, and comfortable speed of walking.56–58 The economy of mobility is a measurement of submaximal oxygen uptake (submax VO2) for a given speed.59,60 A decline in functional performance may be evidenced by an increase in submax VO2 for walking.61 This change in the economy of mobility may be indicative of an abnormal gait pattern.61 Some researchers have reported no gender differences for economy of mobility,62–64 whereas others suggest that men are more economical or have lower-energy costs than women at the same work.65–67 Age-related declines in the economy of mobility also have been reported in the literature, with differing results. Some researchers reported that older adults were less economical than younger adults while walking at various speeds.59,68,69 Conversely, economy of mobility appears to be unaffected by aging for individuals who maintain higher levels of physical activity.70–72
Some authors have claimed that a limb-length discrepancy leads to mechanical and functional changes in gait73 and increased energy expenditure.74 Intervention has been advocated for limb-length discrepancies of less than 1 cm to discrepancies greater than 5 cm,73–75 but the rationale for these recommendations has not been well defined, and the literature contains little substantive information regarding the functional significance of these discrepancies.76 For example, Gross found no noticeable functional or cosmetic problems in a study of 74 adults who had less than 2 cm of discrepancy and 35 marathon runners who had as much as 2.5 cm of discrepancy.75
For gait to be efficient and to conserve energy, the COG must undergo minimal displacement:
Any displacement that elevates, depresses, or moves the COG beyond normal maximum excursion limits wastes energy.
Any abrupt or irregular movement will waste energy even when that movement does not exceed the normal maximum displacement limits of the COG.
To minimize the energy costs of walking, the body uses a number of biomechanical mechanisms. In 1953, Saunders et al.79 proposed that six kinematic features—the Six Determinants—have the potential to reduce the energetic cost of human walking. The six determinants are as follows:80
Lateral displacement of the pelvis: To avoid significant muscular and balancing demands, the pelvis shifts side to side (approximately 2.5–5 cm or 1–2 in) during the gait cycle in order to center the weight of the body over the stance leg. If the lower extremities dropped directly vertical from the hip joint, the COG would be required to shift 3–4 in to each side to be positioned effectively over the supporting foot. The combination of femoral varus and anatomical valgum at the knee permits a vertical tibial posture with both tibias in close proximity to each other. This narrows the step width to 5–10 cm (2–4 in) from heel center to heel center, thereby reducing the lateral shift required of the COG toward either side.
Pelvic rotation: The rotation of the pelvis normally occurs about a vertical axis in the transverse plane toward the weight-bearing limb. The total pelvic rotation is approximately 4 degrees to each side.18 Forward rotation of the pelvis on the swing side prevents an excessive drop in the body’s COG. The pelvic rotation also results in a relative lengthening of the femur by lessening the angle of the femur with the floor, and thus step length, during the termination of the swing interval.81
The vertical displacement of the pelvis: Vertical pelvic shifting keeps the COG from moving superiorly and inferiorly more than 5 cm (2 in) during normal gait. Due to the shift, the high point occurs during midstance, and the low point occurs during initial contact. The amount of vertical displacement of the pelvis may be accentuated in the presence of a leg-length discrepancy, fusion of the knee, or hip abductor weakness, the latter of which results in a Trendelenburg sign. The Trendelenburg sign is said to be positive if, when standing on one leg, the pelvis drops on the side opposite to the stance leg. The weakness is present on the side of the stance leg—the gluteus medius is not able to maintain the COG on the side of the stance leg.
Knee flexion in stance: Knee motion is intrinsically associated with foot and ankle motion. At initial contact before the ankle moves into a plantarflexed position, and thus is relatively more elevated, the knee is in relative extension. Responding to a plantarflexed posture at loading response, the knee flexes. Midstance knee flexion prevents an excessive rise in the body’s COG during that period of the gait cycle. If not for the midstance knee flexion, the COG’s rise during midstance would be larger, as would its total vertical displacement. Passing through midstance as the ankle remains stationary with the foot flat on the floor, the knee again reverses its direction to one of extension. As the heel comes off the floor in terminal stance, the heel begins to rise as the ankle plantarflexes and the knee flexes. In preswing, as the forefoot rolls over the metatarsal heads, the heel elevates even more as further plantarflexion occurs, and flexion of the knee increases.
Ankle mechanism: For normal foot function and gait, the amount of ankle joint motion required is approximately 10 degrees of dorsiflexion (to complete midstance and begin terminal stance) and 20 degrees of plantarflexion (for full push-off in preswing). At initial contact, the foot is in relative dorsiflexion due to the muscle action of the pretibial muscles and the triceps surae. This muscle action produces a relative lengthening of the leg, resulting in a smoothing of the pathway of the COG during stance phase.
Foot mechanism: The controlled lever arm of the forefoot at preswing is particularly helpful as it rounds out the sharp downward reversal of the COG. Thus, it does not reduce a peak displacement period of the COG as the earlier determinants did but rather smooths the pathway. An adaptively shortened gastrocnemius muscle may produce movement impairment by restricting normal dorsiflexion of the ankle from occurring during the midstance to heel raise portion of the gait cycle. This motion is compensated for by increased pronation of the subtalar joint, increased internal rotation of the tibia, and resultant stresses to the knee joint complex.
Joint Motions
During the gait cycle, the swing of the arms is out of phase with the legs. As the upper body moves forward, the trunk twists about a vertical axis. The thoracic spine and the pelvis rotate in opposite directions to each other to enhance stability and balance. In contrast, the lumbar spine tends to rotate with the pelvis. In addition, the pelvis tilts anteriorly and posteriorly (Fig. 6-3). The shoulders and trunk rotate out of phase with each other during the gait cycle.82 Unless they are restrained, the arms tend to swing in opposition to the legs, the left arm swinging forward as the right leg swings forward, and vice versa.5 When the arm swing is prevented, the upper trunk tends to rotate in the same direction as the pelvis, producing an awkward gait.
FIGURE 6-3 Pelvic motion during normal gait.
Maximum flexion of both the elbow and the shoulder joints occurs at the initial contact interval of the opposite foot, and maximum extension occurs at the initial contact of the foot on the same side.86
Although the majority of the arm swing results from momentum, the pendular actions of the arms are also produced by gravity and muscle action.5,87
The posterior deltoid and teres major appear to be involved during the backward swing.
The posterior deltoid serves as a braking mechanism at the end of the forward swing.
The middle deltoid is active in both the forward and the backward swing, perhaps to prevent the arms from brushing against the sides of the body during the swing.
The motions that occur at each of the lower extremity joints during the stance phase of the gait cycle are outlined in Table 6-2 and Figure 6-4.
TABLE 6-2 | Joint Motions in the Lower Extremities During Gait |
Phase | Hip | Knee and Tibia | Ankle and Foot |
Initial contact | Hip begins to move toward extension from a position of 20–40 degrees of flexion; slight adduction and external rotation Reaction force anterior to hip joint creating flexion moment moving toward extension | Knee positioned in full extension before initial contact but flexes as heel makes contact Tibia positioned in slight external rotation Reaction force behind knee rapidly increases causing flexion moment | Foot positioned in supination (rigid) at heel contact, with the ankle moving into plantarflexion Reaction forces behind the joint axis; plantarflexion moment at heel strike |
Loading response | Hip moves into extension, adduction, and internal rotation. Flexion moment | Knee in 20 degrees of knee flexion, moving toward extension Tibia into internal rotation Flexion moment | Foot positioned in pronation to adapt to support surface, with the ankle moving from plantarflexion to dorsiflexion over a fixed foot Maximum plantarflexion moment; reaction force is beginning to shift anteriorly, producing a dorsiflexion moment |
Midstance | Hip moves through neutral position with the pelvis rotating posteriorly Reaction force now posterior to hip joint creating extension moment | Knee in 15 degrees of flexion, moving toward extension Tibia in neutral rotation Maximum flexion moment | Foot in neutral and ankle in 3 degrees of dorsiflexion Slight dorsiflexion moment |
Terminal stance | Hip positioned in 10–15 degrees of hip extension, abduction, and external rotation Extension moment decreases after double-limb support begins | Knee in 4 degrees of flexion, moving toward extension Tibia in external rotation Reaction forces moving anterior to the joint; extension moment | Foot in supination becoming rigid for push-off Ankle in 15 degrees dorsiflexion toward plantarflexion Maximum dorsiflexion moment |
Preswing | Hip moving toward 10 degrees of extension, abduction, and external rotation Continued decrease of extension moment | Knee moving from near full extension to 40 degrees of flexion. Tibia in external rotation Reaction forces moving posterior to knee as knee flexes; flexion moment | Foot in supination and ankle in 20 degrees of plantarflexion Dorsiflexion moment |
Data from Giallonardo LM. Gait. In: Myers RS, ed. Saunders Manual Physical Therapy Practice. Philadelphia, PA: WB Saunders; 1995:1108–1109. |
FIGURE 6-4 Joint kinematics in the horizontal plane during gait.
Hip
The function of the hip is to extend the leg during the stance phase and flex the leg during the swing phase (Table 6-2). Although movement of the hip during gait predominately occurs in the sagittal plane, the hip motion also occurs in the frontal and transverse planes during the gait cycle. During swing, the hip flexes to advance the limb, while, during stance, the hip extends to move the trunk forward until toe-off.88
The hip flexes and extends once during the gait cycle, with the limit of flexion occurring at the middle of the swing phase, and the limit of extension being achieved before the end of the stance phase (Table 6-2). The hip goes through a total excursion of 40–50 degrees during stance, with maximum hip flexion of 30–35 degrees occurring just before the initial contact, at about 85% of the gait cycle. After maximum hip flexion has been obtained, there is often a small movement toward extension (i.e., the hip become slightly less flexed) as the leg adjusts for placement or prepositioning of the foot just prior to initial contact.88 Maximum extension of approximately 10–20 degrees is reached near toe-off at approximately 60% of the cycle.10,40,41,89 The ligaments of the hip helps to stabilize the joint in extension.
In the coronal plane, the hip is slightly abducted at initial contact before hip adduction occurs throughout early stance as the limb is loaded, and the body is supported and reaches a maximum at 40% of the cycle.89 Much of the hip adduction is a result of the pelvic angle so that adduction during stance occurs, in part, due to the dropping of the pelvis on the contralateral unsupported limb. The hip then moves into abduction as the pelvis levels out, and the body progresses over the stance limb, reaching maximum abduction shortly after toe-off.88 The total ROM is near 15 degrees, with equal amounts of abduction and adduction. During the swing phase, hip adduction totaling 5–7 degrees occurs in early swing phase, which is followed by slight hip abduction at the end of the swing phase, especially if a long stride is taken.10,40,41,89
The hip rotates internally and externally approximately 40–45 degrees in the transverse plane during a normal stride, beginning in approximately 10 degrees of external rotation at initial contact.10 The hip begins to internally rotate during the loading response to approximately 5 degrees of internal rotation. Maximum internal rotation occurs around the time of initial contact on the contralateral side.88 The hip externally rotates during the swing phase, with maximal external rotation occurring in terminal swing.40
The greatest force on the hip occurs during midstance. Painful hip, knee, or ankle conditions cause this interval to be shortened to decrease the time spent in the painful position.
Knee
The knee flexes twice and extends twice during each gait cycle: once during weight bearing and once during NWB.
Normal sagittal plane excursion of the knee joint during a gait cycle is approximately 60 degrees with the knee near full extension at initial contact and midstance. At initial contact during normal gait, the GRF initially passes anterior to the knee joint and gives rise to an external knee extension moment, which is counterbalanced by an internal knee flexion moment created by the hamstrings and passive posterior knee structures.9 Immediately after initial contact, the GRF passes behind the knee joint causing an external knee flexion moment as the knee flexes to about 20 degrees during the loading response interval, which acts as a shock-absorbing mechanism. After midstance, the GRF passes in front of the knee again as the knee begins to extend until toe-off and, as the heel rises during the terminal stance interval, the knee is almost fully extended, but flexes again as the swing phase begins. The GRF does not act upon the swing limb. The flexion occurs so that the lower limb can be advanced during the swing phase with a minimum vertical displacement of the COG. The knee then continues to flex as the leg moves into the swing phase. During initial to midswing, the knee continues to flex to a maximum of 60 degrees, before extending again prior to initial contact.28 During terminal swing, the hamstrings act to slow the progression of the tibia in preparation for contact with the ground. Although movements in the frontal and transverse planes can occur at the knee during gait, the magnitude of the excursion is much smaller, making these motions harder to analyze visually. In individuals without joint pathology, there is very little movement in the frontal plane, although there is slight knee varus excursion during the loading response of approximately 4 degrees of tibial abduction range relative to the femur during stance phase.92 The motion of the knee in the transverse plane has been termed the screw home or locking mechanism and is considered a key element in knee joint stability (see Chapter 20).
In normal walking, about 60 degrees of knee flexion is required for adequate clearance of the foot during the swing phase. This peak flexion is required immediately after toe-off because at that point in the gait cycle, the toe is still pointed toward the ground.18
The arthrokinematics involved during the loading response include an anterior gliding of the femoral condyles, which serves to “unlock” the knee. This forward gliding is controlled by the passive restraint of the posterior cruciate ligament (PCL) and by the active contraction of the quadriceps muscles.
A loss of knee extension, which can occur with a flexion deformity, results in the hip being unable to extend fully, which can alter the gait mechanics. Patients with patellofemoral dysfunction demonstrate less knee flexion than normal during the stance phase of gait, combined with increased external rotation of the femur during the swing phase.49 Excessive compensatory internal rotation of the femur of the weight-bearing leg during the stance phase may result in abnormal stresses being placed on the patellofemoral joint.49
Ankle and Foot
Ankle joint motions during the gait cycle occur primarily in the sagittal plane and are associated with balance and forward progression. During normal gait, the initial contact with the ground is made by the heel, with the ankle in a relatively neutral or slightly dorsiflexed position, the heel slightly inverted, and the subtalar joint slightly supinated.105. In individuals with poor control of dorsiflexion (e.g., in cases of hemiplegia), the initial contact is made with the lower part of the heel and forefoot simultaneously. This is usually accompanied by a toe drag during the swing phase. A knee flexion contracture or spasticity may cause the same alteration. Similarly, if the quadriceps are weak, the patient may extend the knee by using the hand or may hit the heel hard on the ground to whip the knee into extension. If heel pain occurs at initial contact, this pain may cause increased flexion of the knee, with early plantarflexion to relieve the stress or pressure on the painful tissues.10
The immediate impact of the initial contact is taken through the lateral tubercle of the calcaneus, a structure unique to humans that is designed to tolerate the shock via the calcaneal fat pad. The calcaneus quickly moves into approximately 5 degrees of valgus as the limb is loaded to dissipate the high GRF that is generated at impact. During the loading response, the ankle moves from neutral dorsiflexion at initial contact to about 5–8 degrees of plantarflexion at midstance. During the loading response interval, the valgus displacement of the calcaneus within the frontal plane induces pronation at the subtalar joint, which in turn results in external rotation of the calcaneus and internal rotation of the talus within the transverse plane.105
The subtalar joint moves into pronation from initial contact to midstance, then rapidly inverts and supinates during terminal stance (after 50% of stance) and peaks near 90% of stance. The pronation of the subtalar joint unlocks the foot and allows maximal ROM of the midtarsal joint, which brings the articulating surfaces of the cuboid and navicular to a position relatively parallel to the weight-bearing surface and allows the forefoot to become supple.107,108 This increase in midtarsal joint mobility enhances the foot’s ability to adapt to the support surface. Abnormal responses during this interval include excessive, or no knee motion, as a result of weak quadriceps, plantarflexor contractures, or spasticity.10
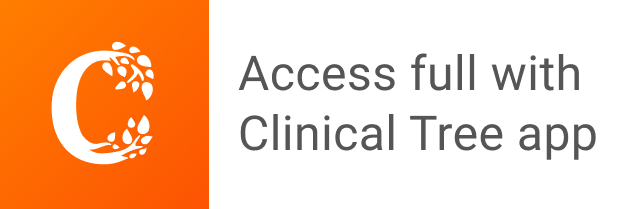