Figure 4.1 Interrelationship of the three key properties of inspiratory muscles: pressure (strength), flow (velocity) and power (strength × velocity). Squares = pressure / flow; circles = pressure / power. (Adapted from McConnell AK, 2011. Breathe strong, perform better. Human Kinetics, Champaign, IL, with permission.)
Inspiratory muscle adaptations to resistance training
The most widely studied muscles are those of inspiration, the functional properties of which have been characterized extensively. As was described above, inspiratory muscle training (IMT) elicits hypertrophy and improvements in strength, and the reader is referred elsewhere for systematic review of the extensive evidence base drawn from both patients and healthy people (Geddes et al, 2008; Gosselink et al, 2011; Hajghanbari et al, 2012; Illi et al, 2012; Plentz et al, 2012; Smart et al, 2012). When IMT is undertaken using moderate loads (~ 60% of MIP) that allow rapid muscle shortening, improvements in strength are accompanied in healthy people by increases in maximal shortening velocity (peak inspiratory flow rate) and maximal power (Tzelepis et al, 1994; Tzelepis et al, 1999; Romer & McConnell, 2003). Moderate loads can typically be sustained for ~ 30 breaths, and have also been shown to improve endurance significantly in healthy people (Caine & McConnell, 1998a). In most studies of resistance IMT a placebo has been used, which typically consists of 30 to 60 breaths at a load of 15% of MIP. The latter has been used repeatedly as an IMT placebo in healthy people as it creates a perceptible resistance, but does not elicit any significant changes in inspiratory muscle function (Volianitis et al, 2001; Romer et al, 2002a; Romer et al, 2002b; Bailey et al, 2010; Brown et al, 2011; Turner et al, 2011; Turner et al, 2012).
Figure 4.2 illustrates the significant changes in strength, peak inspiratory flow and power induced by different IMT regimens in healthy people. It is clear that different types of training induce changes in different functional properties, and this is explored in more detail in Chapter 5 (section on ‘Specificity’), which describes the specificity principle of training, i.e., muscles adapt according to the nature of the training stimulus. For example, it is clear from Figure 4.2 that high-pressure training (maximum static muscle contractions) improves strength, but not velocity of shortening. The opposite was true for high-flow training (unloaded maximal inhalations). No such changes are observed after placebo IMT.
Figure 4.2 Changes in pressure (strength), flow (velocity) and power (strength × velocity) in response to different training stimuli. Dotted lines = post-training; straight lines= pressure / velocity; curved lines = pressure / power. (Data derived from Romer LM, McConnell AK, 2003. Med. Sci. Sports Exerc. 35, 237–244. Adapted from McConnell AK, 2011. Breathe strong, perform better. Human Kinetics, Champaign, IL, with permission.)
As mentioned earlier, strength training can also improve endurance. There is no single accepted method of assessing endurance, but, borrowing the methods used to assess whole-body exercise tolerance, IMT improves inspiratory muscle endurance measured using tests employing continuous incremental and fixed-intensity loading, as well as tests requiring the maintenance of maximal hyperventilation (see below and Ch. 6, section ‘Assessment of respiratory muscle function’). In addition, an improvement in endurance after IMT can be implied from the absence or attenuation of fatigue following a task that had previously induced fatigue, which also occurs after IMT in healthy people (Romer et al, 2002d).
Expiratory muscle adaptations to resistance training
Expiratory muscle training (EMT) has been much less widely studied, but there is good-quality evidence, primarily from patients, that maximal expiratory pressure (MEP) improves significantly in response to specific EMT (19–56%) (Smeltzer et al, 1996; Sapienza et al, 2002; Weiner et al, 2003b; Baker et al, 2005; Chiara et al, 2007; Mota et al, 2007; Kim et al, 2008; Roth et al, 2010). In a particularly comprehensive study on patients with COPD, IMT, EMT and EMT + IMT were studied in three separate, matched groups of patients as well as a fourth placebo control group (Weiner et al, 2003a); this study is unique in that an identical training device and training regimen was used for all of the training. In addition, the IMT and EMT groups received placebo training immediately after their real training session in order to mimic the experience of the EMT + IMT group, who undertook EMT followed immediately by IMT. Following 3 months of IMT the MIP improved significantly by 25%, whereas following EMT the MEP improved significantly by 20%. In the group that undertook EMT + IMT, both MIP and MEP improved significantly by 33%, suggesting that there may be a small potentiation of the training effect when EMT is followed by IMT. Indeed, the IMT and EMT groups both showed small, non-significant increases in maximal pressures for the placebo phase of their training (~ 5%), suggesting a small training effect. In addition, expiratory endurance performance (breathing against an incremental pressure load) improved significantly by 31% following EMT, and significantly by 25% following EMT + IMT, whereas inspiratory endurance improved significantly by 25% following IMT, and significantly by 33% following EMT + IMT. Collectively, these data suggest that, in patients with COPD, expiratory muscles respond in an identical manner to inspiratory muscles when the same training stimulus is applied.
A caveat that is worthy of mention is the ‘purity’, or otherwise, of EMT. A number of studies on patients purporting to undertake EMT have documented improvements in MIP as well as MEP (Roth et al, 2010). On the face of it, this might imply a placebo effect in both groups; however, the effect has been demonstrated in placebo-controlled trials in which there were no significant changes in the placebo groups (Roth et al, 2010). Furthermore, a study that examined the acute responses of inspiratory and expiratory muscles to expiratory loading in healthy people found that it induced a significant decrease in both MIP and MEP, i.e., fatigue (Taylor & Romer, 2009). In other words, expiratory loading also loads the inspiratory muscles. This presumably occurs because of the requirement for inspiratory muscle activation during forceful expiratory efforts. In healthy people, this ‘contamination’ of the training stimulus does not appear to be present during inspiratory loading and IMT, where there is no change in MEP (Romer et al, 2002b; Griffiths & McConnell, 2007), but responses are more variable in patients. For example, patients with spinal cord injury and renal failure show no change in MEP after IMT (Rutchik et al, 1998; Silva et al, 2011), but patients with CHF and multiple sclerosis show a significant increase (Cahalin et al, 1997; Klefbeck & Hamrah Nedjad, 2003). It is possible that these discrepancies are explained by variations in the baseline strength of expiratory muscles and the extent to which they are recruited to lower end-expiratory lung volume during IMT.
Adaptations to simultaneous inspiratory and expiratory muscle resistance training
One might predict that combining IMT and EMT within the same breath cycle (concurrent IMT and EMT) would result in improvements in function in both muscle groups. However, the results of two studies in healthy people that have implemented concurrent training suggest that this approach impairs training responses. For example, a study in young swimmers found no significant change in MIP or MEP despite a strenuous, progressive training regimen (Wells et al, 2005). Similarly, a study on rowers found only modest changes at best in MIP and MEP (Griffiths & McConnell, 2007). Participants generally reported that they found the simultaneous loading of both breath cycles uncomfortable, and that it was impossible to train with maximal effort when both breathing phases are loaded. This may be because of the training stimulus ‘contamination’ effect described in the previous paragraph. However, one study of healthy elderly people has demonstrated significant increases of 22% and 30% in MIP and MEP, respectively, following 8 weeks of concurrent IMT and EMT (Watsford & Murphy, 2008).
Functional adaptations to respiratory endurance training
Pure endurance training of the respiratory muscles is undertaken using a sustained, high-intensity hyperpnoea task (see Ch. 5, ‘Methods of respiratory muscle training’), and has been used much less widely than resistance training, especially in patients. Hyperpnoea training recruits both inspiratory and expiratory muscles simultaneously and, unlike IMT, its nature makes a true placebo difficult to implement. Accordingly, trials have typically included a ‘no training’ control group rather than a placebo group. In healthy people and patients, the training significantly improves the ability to sustain high levels of hyperventilation (an index of endurance) (Scherer et al, 2000; Illi et al, 2012). In healthy people, the training also improves the volume of air that can be respired during a brief maximal burst of hyperventilation, typically 15 seconds (an index of power output) (Verges et al, 2008; Illi et al, 2012). The latter finding is also consistent with improvements in peak velocity of muscle contraction, although there is no direct evidence of this to date. This type of training does not improve the strength of the respiratory muscles in healthy people (Verges et al, 2008; Illi et al, 2012), which is not surprising since the training stimulus required to improve strength must include an increase in the force of muscle contraction (see Ch. 5, section ‘Specificity’). However, in patients with COPD, a small but significant increase in MEP has been shown in one study (Scherer et al, 2000). This may be explained by the presence of expiratory flow limitation in patients with COPD, which creates an expiratory flow resistive load during hyperpnoea. Thus, in the absence of expiratory flow limitation, functional adaptations are typically confined to flow, power and endurance.
Summary
Respiratory muscles respond to training stimuli in the same manner as other skeletal muscles, i.e., by undergoing adaptations to their structure and function that are specific to the training stimulus (see Ch. 5, section ‘Specificity’). Depending upon the training protocol implemented, functional adaptations to respiratory muscle resistance training can include improvement in all four muscle properties, viz., strength, speed of shortening, power output and endurance. Training regimens employing moderate loads (50–60%) sustained to the limit of tolerance (typically 30 breaths) have been shown to generate the widest range of functional benefits (Romer & McConnell, 2003). Expiratory muscle adaptations have been less comprehensively studied, but it is likely that the expiratory muscles respond to training in a similar manner to the inspiratory muscles. Attempts to resistance train both inspiratory and expiratory muscles simultaneously have so far proved impractical, and appear to yield inferior results to loading inhalation and exhalation separately. Specific respiratory muscle endurance training improves speed, power and endurance, but not strength, unless expiratory flow limitation is present thereby creating an expiratory resistive load.
Improving the function of the respiratory muscles is only the first step on the road to a useful clinical intervention. The most important steps on this journey are the changes in functional and clinical outcomes that follow this first step. In the next section, the physiological changes that are stimulated by RMT in healthy young people will be considered. This will be followed by consideration of the disease-specific responses to RMT in a range of clinical conditions.
RESPONSES TO RMT IN HEALTHY PEOPLE
In the first section of this chapter, we considered the structural and functional adaptations elicited by specific training of the respiratory muscles. In Chapter 3 we discovered how breathing limits exercise performance in a range of disease conditions, including performance in sports people. In this section we will see how the muscle adaptations described above overcome the limitations to sports performance described in Chapter 3. This will set the scene for a review of the evidence relating to the clinical benefits of RMT for patients in the final section of this chapter.
To get the most from this chapter, it is desirable (but not essential) to have an understanding of breathing-related limitations to exercise performance in healthy people (as described in Ch. 3). In short, there appear to be two major influences arising from the respiratory pump muscles that can cause athletes to slow down or stop exercise: (1) the perception of breathing effort and (2) the consequences of activation of the group III and IV afferents within the inspiratory muscles. The former makes exercise feel more difficult, and it intensifies as the inspiratory muscles become fatigued; the latter reduces limb blood flow, hastens limb fatigue and exacerbates the perception of limb and whole-body effort.
Logically, making the respiratory muscles stronger and more fatigue resistant should delay or abolish the negative influences of breathing upon exercise tolerance. But what is the scientific evidence supporting this, how big are any improvements and what types of activities are improved? The following description of the published literature is subdivided into two subsections, depending upon the type of performance outcome measure tested: (1) time trials and test of endurance, and (2) tests of anaerobic endurance and sprinting. In addition, this section will consider a study of healthy young people in which the physiological responses to hypoxic conditions were examined before and after RMT. The latter has some bearing on patients with hypoxaemia such as those with COPD. For an overview of the entire evidence base relating to RMT in healthy people, readers are referred to Illi et al’s (2012) systematic review of RMT. This detailed analysis of some 46 original studies of RMT included both strength and endurance RMT, revealing ‘significant improvement in performance after RMT, which was detected by constant load tests, time trials, and intermittent incremental tests, but not by [continuous] incremental tests’. Below is a description of the types of performance tests employed in athletes, as well as a summary of the key papers that have evaluated RMT in athletes.
Influence of RMT upon time trials and tests of endurance
Most of the studies of respiratory muscle training (RMT) in healthy people have examined endurance sports, and two types of exercise tests have been used: fixed-intensity exercise undertaken to the limit of tolerance (Tlim) and time trials. The majority have been randomized placebo-controlled trials; unfortunately, some weaker studies with either a simple no-training control or no control at all have found their way into the literature (Illi et al, 2012). However, only placebo-controlled and controlled trials are considered within this section.
There are no competitive events that require athletes to keep going at the same intensity for as long as they can (a Tlim test), but this type of test does provide an excellent laboratory model for studying the effects of ‘ergogenic aids’ such as RMT (i.e., interventions that purport to improve performance). This is because Tlim tests are extremely sensitive to small physiological improvements, they yield large changes (typically greater than 30%) and they allow physiological and perceptual responses to be studied under identical conditions before and after the intervention. In contrast, the obvious advantage of using a time trial to assess performance is that it simulates a race. However, for this very reason the magnitude of the changes that are typically observed after ergogenic interventions is extremely small, typically less than 5% (Currell & Jeukendrup, 2008). In addition, it is impossible to compare physiological responses in a meaningful way before and after the intervention using a time trial because the exercise conditions are not identical. For example, if performance is enhanced post-RMT, athletes will be working at a greater intensity, which cannot then be compared with the pre-intervention test. Both types of tests have provided useful insights into the effects of RMT.
Typically Tlim tests are conducted at intensities that are just above the lactate threshold. The ability to sustain exercise above the lactate threshold is limited, and the more the intensity of exercise exceeds the lactate threshold the sooner is the onset of exercise intolerance. Studies on RMT have used exercise intensities that can be tolerated for 20 to 40 minutes. In contrast, time trials have varied considerably depending on the sport being studied; for example, studies have used as little as 1 minute for swimming, and as much as 1 hour for cycling.
Studies that have used resistance training of the respiratory muscles (see Ch. 5) have done so using cycling, rowing, swimming and running modalities. In the case of cycling and running, this has been undertaken using both Tlim tests and time trials. For rowing and swimming, only time trials have been used. Table 4.1 summarizes the findings of placebo-controlled studies. Many more studies than those presented have been conducted, but their quality is variable (Illi et al, 2012) so the table is limited to those with robust designs.
Table 4.1
Placebo-controlled studies of the influence of resistance breathing muscle training upon endurance performance
IMT = inspiratory muscle training; EMT = expiratory muscle training; RMT = respiratory muscle training; Tlim = fixed intensity to the limit of tolerance; TT = time trial; O2 = oxygen uptake;
O2max = maximal oxygen uptake; fc = heart rate;
E = minute ventilation; VT = tidal volume; [La]b = blood lactate concentration; RPE = rating of perceived exertion.
*p < 0.05.
Table 4.1 indicates clearly that inspiratory muscle training (IMT) produces statistically significant improvements in performance, but that expiratory muscle training (EMT) does not. As demonstrated statistically by Illi et al (2012), performance improvements occur whether tested using time trials (1.7% to 4.6% improvement for tests lasting 1 to 60 minutes), or Tlim tests (greater than 30% improvement for a 30-minute test). For higher-intensity, shorter-duration Tlim tests, the improvements are smaller (~ 4% for a test lasting less than 4 minutes). This difference in the size of the improvements occurs because of differences in the factors that lead to people stopping exercise and the rate at which these factors accumulate and lead to intolerance (Currell & Jeukendrup, 2008).
Endurance training of the respiratory muscles involves hyperventilation at high levels for prolonged periods (see Ch. 5). Table 4.2 summarizes the controlled and placebo-controlled trials using endurance RMT. Despite the profound difference in the training method, the results are strikingly similar to those for resistance IMT, a finding confirmed statistically by Illi and colleagues (Illi et al, 2012). Typically, Tlim increases by 20% to 50%, whereas a time trial shows ~ 4% improvement. This similarity is quite unlike the response to whole-body endurance and resistance training, which suggests that breathing muscle training taps into a unique and profoundly important mechanism. As was described in the Chapter 3, the most likely candidate mechanism for this effect is an increase in the threshold for activation of the inspiratory muscle metaboreflex.
Table 4.2
Controlled studies of the influence of endurance breathing muscle training upon endurance performance
RMT = respiratory muscle training; Tlim = fixed intensity to the limit of tolerance; TT = time trial; O2 = oxygen uptake;
O2max = maximal oxygen uptake;
E = minute ventilation; [La]b = blood lactate concentration.
* p < 0.05.
It is impossible to separate the inspiratory and expiratory effects of hyperventilation training, but when this has been done for resistance training the independent roles of the two groups of muscles in performance changes become clear (Griffiths & McConnell, 2007): only IMT improves performance. Indeed, adding EMT to IMT during the same breath cycle seems to impair inspiratory muscle responses to IMT (Griffiths & McConnell, 2007) and to negate any performance benefits (Wells et al, 2005), which provides a strong argument against using this approach or, perhaps, undertaking EMT in healthy young people at all.
Tables 4.1 and 4.2 also summarize some of the physiological changes that accompany RMT, and these help to shed some light on the potential mechanisms that do, and do not, lead to the improvement in performance. Specifically, after IMT there are no changes in the ‘usual suspects’ underpinning improved exercise performance after training, viz., maximal oxygen uptake (O2max) and lactate threshold (see Ch. 2). Since breathing does not limit oxygen diffusion (see Ch. 1), we would not expect
O2max to improve. However, the absence of a change in lactate threshold is slightly puzzling given that IMT reduces blood lactate concentrations [La]b during exercise at equivalent intensities (see Tables 4.1 and 4.2). It seems that IMT reduces [La]b, but not the intensity of exercise at which accumulation commences, or indeed the critical power, which is a functional correlate of the lactate threshold (Johnson et al, 2007). The mechanism by which steady state [La]b is reduced without an accompanying change in the lactate threshold is an intriguing one. It is common for different people to have different steady-state lactate concentrations but very similar lactate thresholds, so it is clear that the [La]b per se is not a determinant of the lactate threshold. There is good evidence that IMT increases the metabolic potential of the inspiratory muscles, and thus their ability to consume lactate during submaximal exercise (Brown et al, 2011). One might also hypothesize a lower production of lactate by locomotor muscles due to enhanced oxygen delivery, which could arise because of a reduction in sympathetic vasoconstriction. However, improving oxygen delivery in sub-elite athletes by inhaling an oxygen-rich inspirate does not enhance their lactate threshold (Sadowsky et al, 1995). Thus the most likely explanation for the lower steady state [La]b without a change in the lactate threshold is enhanced consumption of lactate by the inspiratory muscles (Peter Brown & Graham Sharpe, personal communication). However, this does not imply that an improved muscle blood flow is redundant. Enhancing muscle blood flow by manipulating the work of breathing reduces exercise-induced locomotor muscle contractile fatigue (Romer et al, 2006). Furthermore, the removal of muscle metabolites may also influence the central contribution to fatigue (see Ch. 1, ‘Mechanisms of fatigue’).
So far as the factors that do change after IMT are concerned, these include reductions in [La]b, heart rate, and perception of breathing and limb effort, as well as a speeding of oxygen uptake kinetics during heavy exercise. In addition, breathing becomes deeper and slower, as well as more metabolically efficient (Turner et al, 2012). Perhaps most importantly, IMT delays or abolishes the exercise-induced decrease in MIP observed post-exercise, i.e., IMT attenuates inspiratory muscle fatigue (Romer et al, 2002d). These changes are all consistent with the respiratory-related exercise-limiting factors described in Chapter 3. The attenuation of inspiratory muscle fatigue is indicative of an improvement in the functional capacity of the respiratory pump muscles. Since metabolite accumulation contributes to deficits in the ability to produce muscular force at both a peripheral and a central level (see Ch. 2, ‘Mechanisms of fatigue’), the attenuation of inspiratory muscle fatigue is consistent with a reduction and / or delay in both metabolite accumulation and activation of the inspiratory muscle metaboreflex. Activation of this reflex induces vasoconstriction in the locomotor muscles, but IMT has been shown to increase the intensity of inspiratory muscle work required to activate this reflex (McConnell & Lomax, 2006; Witt et al, 2007; Bailey et al, 2010), and to reduce La production by the inspiratory muscles (Brown et al, 2011). As a result, muscle blood flow may be preserved, and limb fatigue attenuated or delayed (McConnell & Lomax, 2006). This mechanism may also explain the reduction in [La]b, and leg effort, as better-perfused locomotor muscles generate less lactate and have lower levels of metabolites to stimulate the group III and IV afferents that contribute to effort perception (Amann et al, 2010) and fatigue (Gandevia, 2001; Amann et al, 2009) (see Ch. 3). In addition, if inspiratory muscle fatigue is attenuated the perception of breathing effort will be reduced, making it possible to maintain a more efficient deep, slow breathing pattern (Turner et al, 2012). In short, the physiological changes point strongly to the ergogenic effect of IMT being underpinned by preservation of limb blood flow and reduction in breathing effort. These effects are arguably even more important in patients, since exercise tolerance is frequently limited by dyspnoea and / or muscle dysfunctions that are exacerbated by impaired blood flow.
In respect of the inspiratory muscle metaboreflex, there is one particular study of IMT that is worthy of detailed scrutiny because it has particular relevance to patients. The study in question not only demonstrated that IMT extends Tlim, but it also demonstrated that oxygen uptake kinetics were hastened during ‘severe’- and ‘maximal’-intensity cycling (Bailey et al, 2010), a finding that has since been confirmed (Brown et al, 2011). The authors’ interpretation was that their observations were due to ‘increased blood flow to the exercising limbs’, and that this arose because of the absence of inspiratory muscle metaboreflex activation post-IMT (Bailey et al, 2010). These findings are especially relevant to patients since the oxygen uptake kinetics of patients are slowed by disease and inactivity (Chiappa et al, 2008a). The speed of this ‘on transient’ of the oxygen uptake kinetics determines the size of the oxygen deficit during exercise, and thus the size of the anaerobic contribution at the onset of exercise (see Ch. 1). Enhancing the amount of energy liberated from aerobic sources minimizes the production of fatiguing metabolic by-products, and reduces the ventilatory requirement for exercise. In patients for whom walking constitutes ‘severe’-intensity exercise, and who are consequently teetering on the edge of the ‘anaerobic abyss’, this effect on oxygen uptake kinetics may be particularly important and make a disproportionate contribution to improving exercise tolerance. Interestingly, reducing the work of breathing using a low-density inspirate (heliox) speeds oxygen uptake kinetics and improves exercise tolerance in patients with COPD (Chiappa et al, 2009); this response is strikingly similar to that observed in healthy people following IMT (Bailey et al, 2010; Brown et al, 2011).
Finally, there has long been speculation that IMT may enhance the mechanical efficiency of breathing, as some studies have shown small (often non-significant) decreases in the oxygen cost of exercise (Romer et al, 2002a; Griffiths & McConnell, 2007; Turner et al, 2011). A recent study found that 6 weeks of IMT (30 repetitions against a load equivalent to 50% of MIP) reduced the oxygen cost of hyperpnoea by 5–12% (Turner et al, 2012). The greatest decrement in oxygen cost was seen at the highest intensity of respiratory muscle work. This finding has important implications for patients in whom disease-related impairments of breathing mechanics increase the oxygen cost of breathing. Since the latter may account for a considerable proportion of available oxygen uptake, reducing this burden may release oxygen for use by other muscle groups, thereby enhancing exercise tolerance.
In summary, the research using time trials and tests of endurance indicates that, for bouts of exercise involving a sustained effort against the clock of 1 to 60 minutes duration, IMT improves performance significantly by 1.7% to 4.6%. Although there is no direct research evidence that similar improvements will be seen during longer events (e.g., marathons or triathlons), the fact that a decline in MIP has been demonstrated after such events means that performance in these events may be limited by breathing-related factors. Accordingly, there is every reason to believe that IMT will improve performance in events lasting more than 1 hour. The mechanisms that underlie the changes in performance described above are applicable universally and have equal, if not greater, relevance to exercise-limited patients. Indeed, Illi and colleagues’ systematic review revealed that the ergogenic effect of RMT was greatest in the least fit participants (Illi et al, 2012).
Influence of RMT upon tests of anaerobic endurance and sprinting
Sprinting is such a brief activity that the benefits of IMT are not immediately obvious. However, the changes that IMT induces in underlying physiology appear to be so fundamental that it is now clear that performance in sprint tasks can also benefit from IMT. This is relevant to patients because the metabolic demands of activities of daily living can be akin to those of a sprint in a young healthy individual. In the previous section we have already discussed the fact that maximal cycling to the limit of tolerance is extended significantly following IMT, and that this was accompanied by a significant speeding up of oxygen uptake kinetics (Bailey et al, 2010; Brown et al, 2011). Repeated sprinting may not be something that many patients engage in during their everyday lives, but it is encountered in interval training, which is finding increasing favour within rehabilitation programmes (Mador et al, 2009). Thus, the results of studies on repeated sprinting suggest potential benefits of IMT for tolerance to repeated bouts of anaerobic exercise, such as interval training. Improving the tolerability of training interventions has the benefit of enhancing the potency of the training stimulus, and the resulting performance benefits. Indeed, it has been shown that athletes undertaking a period of IMT prior to a programme of interval training were able to train significantly harder, and showed significantly greater improvements in repeated sprint performance, compared with a group that did not receive prior IMT (Tong et al, 2010). Unfortunately, there was no placebo control within this trial.
In the context of team sports, a sense of increased breathing effort between sprints has a profound influence on a player’s ability to sprint again, and in competition this has implications for the quality of the athlete’s contribution to the match or game. For this reason, the first study to examine the effect of IMT on sprinting used perceived rate of recovery during continuous bouts of repeated sprinting (Romer et al, 2002b). The expectation was that IMT would reduce breathing effort between sprints and delay the onset of inspiratory muscle fatigue, thereby making the participants feel as if they had recovered more quickly. However, because the sprint was very brief (3.2 seconds) and punctuated with periods of recovery, there was no expectation that actual sprint performance would improve. These expectations were confirmed; after 6 weeks of IMT (30 repetitions against a load equivalent to 50% of MIP) the athletes showed a significantly faster rate of recovery compared with baseline and placebo, but no change in sprint performance. The potential relevance of these findings for a patient who is limited acutely by dyspnoea is that IMT may reduce the duration that they must spend ‘catching their breath’; this hypothesis awaits evaluation.
Using a slightly different approach, two controlled studies (one of which was placebo controlled) have explored the benefits of IMT to the ability to sustain repeated sprinting, with gradually escalating sprint speed, and short active recovery breaks between sprints (Yo-Yo test) (Bangsbo et al, 2008). Performance in a test of this kind is influenced by both effort perception and factors related to limb blood flow, such as oxygen delivery and metabolite removal. As has been explained previously, both of these factors are potentially influenced by IMT, via its hypothesized effects upon breathing effort and activation of the inspiratory muscle metaboreflex. After 5–6 weeks of IMT (30 repetitions against a load equivalent to 50% of MIP), both studies found that performance in the Yo-Yo test improved significantly by ~ 17% (Tong et al, 2008; Nicks et al, 2009). Accompanying the improvement in performance were significant reductions in perception of breathing and whole-body effort, as well as markers of metabolic stress. In a more recent study, the distribution of blood flow to the legs and respiratory muscles during repeated sprinting has been studied (Tong et al, 2012). As the sprint test progressed, minute ventilation showed a progressive increase and, at the point where respiratory compensation for the developing metabolic acidosis commenced, there was a clear reduction in the oxygenation of both the respiratory and leg muscles. The timing of the two events was correlated significantly. These data are consistent with activation of the inspiratory muscle metaboreflex by the escalating ventilatory demand, followed by vasoconstriction of both the respiratory and leg muscle vasculature. Thus, as suggested for endurance exercise, IMT may improve performance during repeated sprinting by increasing the intensity of respiratory muscle work required to activate the metaboreflex, thereby preserving limb blood flow (McConnell & Lomax, 2006; Witt et al, 2007; Bailey et al, 2010).
Most recently, the effects of combining IMT with a specific inspiratory muscle warm-up (see Ch. 6) have been tested in a placebo-controlled trial on semi-professional football players (Lomax et al, 2011). The same IMT protocol and repeated sprint test were used as in previous studies; after 4 weeks of IMT, sprint performance increased significantly by 12%. At baseline, the warm-up increased sprint performance significantly by 5–7% in both groups, which is consistent with previous findings (Tong & Fu, 2006). When an inspiratory muscle warm-up was added after IMT, performance increased significantly by a further 2.9%. Thus, the benefit of the inspiratory muscle warm-up and IMT were additive (14.9%).
In summary, IMT was once thought to be of benefit only for performance in activities dominated by aerobic metabolism. However, studies now suggest that IMT is more versatile, and that the fundamental nature of the underlying mechanisms makes it an effective tool for enhancing tolerance to both prolonged moderate-intensity exercise and brief intense exercise. The similarity between the physiological changes observed following IMT during sprint and endurance exercise in healthy people is to be expected, given that IMT is probably operating via the same underlying mechanisms in both situations, i.e., attenuation of breathing effort and enhancement of limb blood flow.
Influence of RMT under hypoxic conditions
The addition of an hypoxic drive to breathe during exercise places a large additional demand upon the respiratory muscles. Therefore, the potential benefits of RMT are arguably even greater to people exercising in an hypoxic environment, or those who have hypoxaemia due to the effects of disease. To date, only one placebo-controlled study has assessed the influence of IMT on exercise tolerance and physiological responses to exercise in hypoxia (Downey et al, 2007). The results of the randomized controlled study were both impressive and surprising. The IMT group trained for 40 breaths, 5 days per week at a load of ~ 50% of MIP, whilst the placebo group used the same regimen at a load of 15% of MIP. The hypoxic environment simulated an altitude of ~ 3500 metres (~ 12 000 feet), which was sufficient to reduce arterial saturation to ~ 93% at rest and ~ 77% at end of exercise. Following 4 weeks of IMT, minute ventilation, cardiac output and oxygen uptake of hypoxic treadmill exercise were reduced significantly in the IMT group by 25%, 14% and 8–12% respectively. Despite the lower minute ventilation, arterial oxygen saturation (SaO2) and lung-diffusing capacity were increased by 4% and 22%, respectively. Perceived exertion and dyspnoea were also reduced significantly. No such changes were observed in the placebo group. The changes in minute ventilation and SaO2 appear to be completely at odds with one another, as an increase in SaO2 normally requires an increase in minute ventilation. The clue to resolving this paradox resides in the improvement in the diffusing capacity of the lungs, which can increase only if the lung diffusion surface area increases. The pulmonary vasculature is responsive to muscle metaboreflex activation (Lykidis et al, 2008). Thus, if IMT were to delay or abolish activation of the inspiratory muscle metaboreflex, it is conceivable that blood flow would be preserved in the pulmonary circulation. Should this occur, an increase in diffusion surface area would result, thereby increasing SaO2. The effect of IMT upon SaO2 in hypoxic conditions has been confirmed under resting conditions in a randomized controlled study of military personnel during an expedition to the Nepali Himalayas (Lomax, 2010). At altitudes of 4880–5550 metres, the resting SaO2 was 6% higher in the IMT group compared with the control group. This protective effect upon SaO2 was not observed at 1400 metres.
High altitude is the only environment where the lungs limit oxygen transport in healthy people. However, there are many disease states that result in hypoxaemia and pulmonary vasoconstriction, both at rest and during exercise. Under these conditions, patients may be particularly sensitive to an additional vasoconstrictor input to the pulmonary vasculature from the inspiratory muscle metaboreflex. Accordingly, IMT may provide even greater benefits to these patients than those observed in normoxic, healthy people. In the following section, the evidence relating to the influence of RMT upon patients with a range of conditions will be reviewed.
DISEASE-SPECIFIC FUNCTIONAL RESPONSES TO RMT
In most of the literature that is reviewed below, the mode of RMT has been inspiratory resistance training. However, there have been a handful of studies using EMT, or endurance RMT, and these are identified accordingly.
Respiratory disease
Chronic obstructive pulmonary disease
Respiratory muscle training has been studied most extensively in patients with COPD, and the type of intervention implemented has overwhelmingly been inspiratory muscle resistance training (IMT). The first published studies appeared in the late 1970s and the number of studies appearing each year has grown steadily, such that the most recent meta-analysis of this literature included a total of 32 studies (Gosselink et al, 2011); however, these studies represent only the [high-quality] tip of an IMT research iceberg. As was explained in Chapter 3, the rationale for IMT in patients with COPD is the restoration of balance in the demand / capacity relationship of the respiratory pump muscles. Patients with COPD have primary weakness of inspiratory muscles, which is exacerbated by functional weakness. In addition, there is an increased demand for inspiratory muscle work, owing to changes in breathing mechanics and the elevated ventilatory requirement for exercise. The primary exercise-limiting symptom under these conditions is dyspnoea.
The extensive nature of the literature addressing IMT means that there has been a systematic review (Shoemaker et al, 2009) and two meta-analyses (Geddes et al, 2008; Gosselink et al, 2011) published since 2008. Accordingly, it is possible to draw conclusions about the usefulness of IMT in patients with COPD based upon statistical evidence. The three reviews are in agreement, indicating that IMT improves: inspiratory muscle strength (MIP) and endurance, exercise capacity (6-minute walk distance), dyspnoea, and quality of life. Gosselink et al (2011) found the effect sizes of these responses to be ‘medium to large’. In sub-analyses, inspiratory muscle strength training was found to be superior to endurance training for improving exercise capacity and dyspnoea. In addition, patients with the lowest MIP at baseline showed the greatest improvements in MIP and exercise capacity.
An area that has so far received very little attention is the impact of IMT upon the use of healthcare resources. As part of a 12-month placebo-controlled trail of IMT, Beckerman et al (2005) not only noted improvements in MIP, exercise capacity, dyspnoea and quality of life, but also observed significant differences between the IMT group and the placebo group in the number of primary care consultations and days spent in hospital across the intervention. There was also a difference in the number of patients admitted and the total number of admissions, but these failed to reach significance. These data suggest that IMT may reduce the number of exacerbations and hasten recovery from exacerbations. However, further research is needed in order to clarify these potential benefits.
A question that remains unclear at present is the best way to incorporate IMT into a rehabilitation programme. As was described above, there is robust evidence that IMT can be used as a stand-alone intervention to improve inspiratory muscle strength and endurance, exercise capacity, dyspnoea and quality of life. However, it remains unclear whether IMT provides additional improvements when added to a rehabilitation programme that includes whole-body exercise. Nevertheless, based upon the evidence of Lotters et al (2002) and O’Brien et al (2008), addition of IMT to a general exercise programme for patients with inspiratory muscle weakness is recommended by the joint BTS / ACPRC Guidelines (Bott et al, 2009).
A recent systematic review comparing IMT and rehabilitation revealed that, compared with exercise alone, improvement in MIP and one index of exercise tolerance were superior if IMT was added to exercise (O’Brien et al, 2008). Interestingly, when comparing IMT with exercise training, the study also found ‘no significant difference in effect for outcomes of MIP and exercise tolerance among patients with COPD who engage in IMT compared with exercise’ (O’Brien et al, 2008). In other words, IMT appears to improve exercise tolerance as much as exercise. Furthermore, a systematic review of home-based physiotherapy interventions for patients with COPD found that home-based IMT significantly improved dyspnoea (on the Transitional Dyspnoea Index) by 2.36 units compared with the control (Thomas et al, 2010). These findings suggest that IMT is almost certainly a more cost-effective intervention than exercise for improving dyspnoea, as it can be implemented in a domiciliary setting with minimal supervision. It is also pertinent to recall that, in healthy people, implementing IMT prior to whole-body training improved the outcome of the whole-body training (Tong et al, 2010); thus IMT might be considered as ‘pre-habilitation’ for patients awaiting commencement of exercise training.
Expiratory muscle training has received very little attention. To date, there have been only two placebo-controlled studies examining the effect of EMT in patients with COPD: one using specific EMT (Weiner et al, 2003b), and the other comparing responses with EMT, IMT and combined IMT and EMT (Weiner et al, 2003a). In the EMT study, expiratory muscle strength (MEP) and endurance increased significantly, as did exercise capacity. There was no significant change in the sensation of dyspnoea during daily activities. In the second of the two studies (Weiner et al, 2003a), eight patients were assigned to receive EMT, eight received IMT, eight received EMT + IMT and a group of eight was assigned to be a placebo group. There were no changes in the placebo group, but training induced statistically significant, specific increases in MEP and endurance (in the EMT and in the EMT + IMT groups) and in MIP and endurance (in the IMT and EMT + IMT groups). Exercise capacity increased in all three training groups. However, the increase in the IMT and the EMT + IMT groups was significantly greater than that in the EMT group. There was also a decrease in dyspnoea in the IMT and in the EMT + IMT groups, but not in the EMT or placebo groups. It was concluded that the inspiratory and expiratory muscles can be trained specifically to improve both strength and endurance. However, there appears to be no additional benefit gained by combining IMT + EMT, compared with IMT alone.
Finally, there are two specific, less obvious applications of IMT that are worthwhile mentioning. The first relates to the observation that repeated, deep inhalations against an inspiratory load have been found to be twice as effective (as measured by sputum weight) as standardized physiotherapy consisting of postural drainage and the active cycle of breathing technique (Chatham et al, 2004). Thus IMT may also facilitate airway clearance. The second application is in promoting the ability of patients to use inhalers. Dry-powder inhalers (DPI) have been suggested to be more appropriate for patients with coordination difficulties. However, these often have a much higher inherent flow resistance, thus rendering them unusable by very weak patients; one study found that 20% of patients with COPD were unable to generate the required inspiratory flow rate to generate the required flow rate via the Turbohaler® DPI (Weiner & Weiner, 2006). Furthermore, the flow rates achieved through the DPI were correlated with MIP. After the patients had received an 8-week period of IMT, all were able to generate the required flow rate via the Turbohaler®. Thus IMT may be a useful to tool enable and improve DPI use by very weak patients.
In summary, the strength of evidence supporting the efficacy of RMT, and in particular IMT, in patients with COPD has achieved a level where it is possible to recommend IMT as a stand-alone treatment. Improvements in inspiratory muscle strength and endurance, exercise capacity, dyspnoea and quality of life can be expected. Questions remain regarding whether the addition of IMT to exercise training provides any supplemental benefits, but the preliminary indications are that outcomes such as dyspnoea are improved to a greater extent by implementing the two interventions in parallel. The role of EMT is much less clear, owing to a lack of studies. Patients with the weakest inspiratory muscles appear to show the greatest improvements following IMT, but there is no minimum threshold above or below which benefits can, or cannot, be anticipated. Furthermore, acute bouts of loaded breathing may also facilitate airway clearance. For specific recommendations regarding implementation of IMT see Chapters 5 and 6.
Asthma
Inspiratory muscle training has been studied less extensively in patients with asthma than in those who have COPD. As was presented in Chapter 3, the rationale for IMT in patients with asthma is the restoration of balance in the demand / capacity relationship of the respiratory pump muscles, which are overloaded when airway obstruction is present. The primary symptom under these conditions is dyspnoea. To date, there has been one attempt at a Cochrane systematic review (Ram et al, 2003), which included just five studies. The review concluded that there were insufficient data, and further research was required. However, the analysis was able to confirm that IMT yielded significant improvements in MIP. Unfortunately, since 2003 there has been only one further study of IMT in people with asthma. The findings of the available literature are summarized below, and are also placed in a mechanistic context.
A notable feature of airway obstruction in asthma is the large inter-subject variation in the intensity of dyspnoea for a given fall in FEV1 (Lougheed et al, 1993). Furthermore, women appear to experience higher levels of dyspnoea (Wijnhoven et al, 2003), poorer quality of life and more frequent hospital admission than do men (Weiner et al, 2002c). These observations led Weiner and colleagues to reason that the gender difference might be explained, at least partially, by the fact that women have weaker inspiratory muscles than men (Weiner et al, 2002c). Accordingly, the influence of gender and inspiratory muscle strength (MIP) was examined by comparing the MIP, perception of dyspnoea to threshold loads, and bronchodilator consumption of 22 male and 22 female asthmatic patients with mild-to-moderate bronchoconstriction (FEV1 > 60% of predicted) (Weiner et al, 2002c). For the same FEV1 (% of predicted), the women had significantly weaker inspiratory muscles, whilst dyspnoea during loaded breathing and β2-agonist consumption was significantly higher than in the men. The women were divided randomly into two groups: half received IMT, and the remainder received sham training. After 20 weeks of IMT, the MIP of the women (+ 42%) matched that of the men. Accompanying this change was a reduction in the dyspnoea during loaded breathing and the β2-agonist consumption of the women in the IMT group, compared with that of the placebo group. Furthermore, after IMT, dyspnoea and β2-agonist consumption were no longer different to those in the men.
The strong interrelationship between MIP, dyspnoea and β2-agonist consumption was confirmed in a study designed to examine their interrelationships specifically (Weiner et al, 2002b). There was no correlation between baseline measures of MIP and intensity of dyspnoea during loaded breathing in a sample of 30 patients. However, after IMT both the total medications use and the perception of dyspnoea during loaded breathing showed a direct, quantitative relationship with the increase in MIP. Indeed, 93% of the change in β2-agonist consumption was explained by the change in MIP. These findings support a role for the absolute strength of the inspiratory muscles in determining the consumption of medication, and dyspnoea.
Critics will argue that changes in dyspnoea during loaded breathing are not the same as exertional dyspnoea, and they would be correct. However, there is evidence that exertional dyspnoea is also attenuated after IMT in people with asthma (12.4% reduction), and after as little as 3 weeks of IMT (McConnell et al, 1998). Most recently, a comprehensive study of IMT using a matched double-blind placebo-controlled design confirmed that exertional dyspnoea was reduced (by 16%) after 6 weeks of IMT (Turner et al, 2011); it also noted further benefits, which included an improvement in exercise tolerance, attenuation of exercise-induced decrease in MIP, and a lower oxygen cost of exercise (reduced by 12% at Tlim). The latter was presumed by the authors to derive from improvements in the mechanical efficiency of breathing, which derives some support from their later finding that in healthy people the oxygen cost of voluntary hyperpnoea is reduced by as much 12% after IMT (Turner et al, 2012).
An impressive feature of studies of IMT in patients with asthma is the reduction in β2-agonist consumption that accompanies IMT. In the three studies in which this has been examined (Weiner et al, 1992; Weiner et al, 2000; Weiner et al, 2002c), this reduction has ranged from 38% to 78%, being greatest in those with the highest baseline consumption. Thus, IMT appears to be particularly helpful for patients with high levels of dyspnoea and β2-agonist consumption. Furthermore, a double-blind placebo-controlled trial of IMT conducted over 6 months also observed significant improvements in lung function, asthma symptoms, hospitalizations for asthma and absence from school or work (Weiner et al, 1992).
However, a note of caution is warranted at this point, because there are a small group of patients with asthma for whom further reductions in the intensity of dyspnoea sensation may be life threatening. According to one study, around 26% of patients with asthma have abnormally low perceptions of dyspnoea (Magadle et al, 2002); this was associated with low consumption of medication, increased emergency department visits, hospitalizations, near-fatal asthma attacks, and deaths during follow-up (Magadle et al, 2002). A second study confirmed the association between near-fatal asthma and low perceptions of dyspnoea, as well as blunted hypoxic sensitivity (Kikuchi et al, 1994). It would therefore be inappropriate to implement IMT in this subgroup. However, the available evidence suggests that patients with normal or high sensation of dyspnoea do not become desensitized to bronchoconstriction following IMT. Weiner et al (2000) noted that IMT did not result in exaggerated ablation of dyspnoea, and concluded that IMT was safe, at least for use in patients with mild asthma.
In summary, the strength of evidence supporting the efficacy of IMT in patients with asthma has not yet achieved that of COPD. This is primarily because of the small number of studies available to date. There is some evidence that dyspnoea and use of medication are greatest in those with the weakest inspiratory muscles (lowest MIP). Preliminary evidence also suggests that, irrespective of MIP, IMT induces improvements in inspiratory muscle strength, exercise capacity, dyspnoea and use of medication. Patients with abnormally low dyspnoea perception are unsuitable candidates for IMT, but there appears to be no evidence that inspiratory muscle weakness is a prerequisite to improvements following IMT. Furthermore, acute bouts of loaded breathing may also facilitate airway clearance. For specific recommendations regarding implementation of IMT see Chapters 5 and 6.
Bronchiectasis
Bronchiectasis is a chronic lung disease that is not normally included within the umbrella of COPD. However, the functional manifestations of bronchiectasis have similarities with those of COPD, including airway obstruction, which leads to detrimental changes in breathing mechanics, attendant exertional dyspnoea and exercise intolerance (Neves et al, 2011). Patients with moderate-to-severe bronchiectasis also have slight respiratory muscle weakness (Moran et al, 2010). It is therefore reasonable to hypothesize that responses to IMT in patients with bronchiectasis would be similar to those of patients with COPD.
To date there have been just two studies in which patients with bronchiectasis have been subjected to IMT. In the first study, moderate-intensity IMT (15 minutes breathing against 30–60% of MIP) was undertaken for 8 weeks in combination with a programme of physical exercise (EX + IMT). The responses of this IMT group were compared with those of a group who received exercise and sham IMT (EX + sham), as well as a control group (Newall et al, 2005). There was a significant increase in MIP in both training groups (18% and 33% for exercise and EX + IMT, respectively), but the increase was not significantly different between the groups. Exercise tolerance improved significantly in both training groups during a treadmill Tlim test and in an incremental shuttle walking test; but the changes were not significantly different between groups. Thus adding IMT to exercise training in these patients did not result in significantly greater improvements in MIP of exercise tolerance. However, a key finding of this study was that, 3 months after cessation of the interventions, exercise tolerance was maintained in the EX + IMT group but not in the EX + sham group. In addition, the EX + IMT group was the only group to show an improved quality of life score, which was also maintained after cessation of training. It is noteworthy that the improvements in all parameters were largest in the EX + IMT group, but it is important to bear in mind that the assessment of IMT was made on an improving baseline of function due to the exercise training, which was implemented in both the IMT and sham groups; the effect of this would be to reduce the effect size of the IMT. Finally, the lack of statistically significant difference between EX + sham and EX + IMT may be due to lack of statistical power, due to the small sample size (~ 10 participants per group). The failure to distinguish an additional benefit of adding IMT to exercise has also been observed in patients with COPD, but a recent systematic review concluded that ‘[in COPD] Results showed significant improvements in maximum inspiratory pressure and maximum exercise tidal volume favoring combined IMT and exercise compared with exercise alone’ (O’Brien et al, 2008). Conclusions regarding other outcomes await further data.
In a more recent study (Liaw et al, 2011), a low-intensity IMT regimen (30 minutes breathing against 30–38% of MIP) was evaluated in 13 patients, and compared with 13 patients who did no training at all. Both MIP and MEP increased in the IMT group (39% and 44%, respectively), and although there was a significant increase in 6-minute walk distance (14.8%), this just failed to be significantly different from that of the control group. Interpretation of these data is hampered by lack of statistical power, and the absence of a placebo control. Taken together, the two studies provide preliminary evidence that IMT may elicit similar improvements to those shown in patients with COPD. The joint BTS / ACPRC Guidelines make the following recommendation in relation to IMT in bronchiectasis: ‘Consider the use of inspiratory muscle training in conjunction with conventional pulmonary rehabilitation to enhance the maintenance of the training effect’ (Bott et al, 2009).
Finally, it is worthwhile mentioning that some IMT studies on patients with CF have reported improvements in expectoration immediately after IMT (Asher et al, 1982; Enright et al, 2004); indeed, repeated, deep inhalations against an inspiratory load have been found to be twice as effective (measured by sputum weight) as standardized physiotherapy consisting of postural drainage and the active cycle of breathing technique (Chatham et al, 2004). Thus IMT may also facilitate airway clearance in patients with bronchiectasis.
In summary, interpretation of the evidence relating to the efficacy of IMT in patients with bronchiectasis is hampered by the very small number of studies. Taken together, the two available studies provide preliminary evidence that IMT may elicit similar improvements to those shown in patients with COPD. Further research on IMT as a stand-alone intervention is justified, as the theoretical rationale for IMT in patients with bronchiectasis is very similar to that for patients with COPD. Furthermore, acute bouts of loaded breathing may also facilitate airway clearance. For specific recommendations regarding implementation of IMT see Chapters 5 and 6.
Cystic fibrosis
Although there are a handful of studies of RMT in patients with CF, one of these failed to include a control group (Keens et al, 1977) and one utilized an unreliable method of training (Asher et al, 1982). Of the remaining three, only two studies (de Jong et al, 2001; Enright et al, 2004) met the criteria to be included in a recent systematic review (Reid et al, 2008). Although inevitably limited, this review represents the most up-to-date picture of the influence of IMT upon patients with CF (Reid et al, 2008). Analysis of both studies revealed no significant effects of IMT upon lung function or inspiratory muscle strength (MIP). However, these findings cannot be taken at face value as some important features of the two studies differed. In one study, the training regimen was 20–40% of MIP sustained for 20 minutes (de Jong et al, 2001). This constitutes an endurance training regimen; indeed, endurance increased significantly, but not strength – a finding ascribed by the authors to the low intensity of the training. Accordingly, these data should be interpreted cautiously in respect of the efficacy of IMT. In the other study within the review (Enright et al, 2004), a high-intensity inspiratory muscle-strength-training programme was implemented. This high-intensity training elicited improvements in MIP (18%) and endurance, as well as diaphragm thickness. Furthermore, lung function, incremental cycle performance and psychological status were also improved significantly. Whilst seemingly contradictory, the systematic review of these two studies most likely highlights the differing efficacy of the two types of training implemented.
It is not clear why the fifth study was excluded from the systematic review, but this may be because it was not a randomized trial (Sawyer & Clanton, 1993). However, it was a placebo-controlled trial and therefore merits consideration. After 10 weeks of IMT using a moderate load (50–60% of MIP), there were significant improvements in MIP (13%), lung function and exercise tolerance. The greater training intensity and longer duration of the intervention may explain the discrepancy between this study and that of de Jong et al (2001).
Finally, two IMT studies on patients with CF have reported improvements in expectoration immediately after IMT (Asher et al, 1982; Enright et al, 2004); indeed, repeated deep inhalations against an inspiratory load have been found to be twice as effective (measured by sputum weight) as standardized physiotherapy consisting of postural drainage and the active cycle of breathing technique (Chatham et al, 2004). It has been suggested that the effect is similar to that seen after exercise. This finding may have implications for other patient groups in which airway clearance is problematic.
In summary, interpretation of the evidence relating to the efficacy of IMT in patients with CF is hampered by the very small number of studies. When viewed collectively, the data from placebo-controlled trials suggest that, when moderate- to high-intensity loading is used, MIP improves by 13–18% and is accompanied by a range of clinically significant benefits. Further research on IMT as a stand-alone intervention is justified as there is a good theoretical rationale for IMT in patients with CF (see Ch. 3). Furthermore, acute bouts of loaded breathing may also facilitate airway clearance. For specific recommendations regarding implementation of IMT see Chapters 5 and 6.
Restrictive chest wall disorders
Conditions such as kyphoscoliosis, fibrothorax, thoracoplasty, flail chest and ankylosing spondylitis create a restrictive pulmonary defect in which total respiratory system elastance and resistance are elevated (Donath & Miller, 2009). Furthermore, inspiratory muscle function also tends to be impaired (Lisboa et al, 1985; Cejudo et al, 2009) owing to changes in chest wall and diaphragm configuration.
Given the obvious imbalance in the demand / capacity relationship of the respiratory pump, it is surprising that there has been only one randomized controlled trial of IMT in patients with restrictive chest wall disease (Budweiser et al, 2006). Thirty patients with restrictive lung disorders who were receiving intermittent non-invasive positive-pressure ventilation (NPPV) took part. Half underwent 3 months of endurance RMT (a minimum of 10 minutes of hyperpnoea training), and half sham RMT (incentive spirometry). Surprisingly, endurance RMT induced significant improvements in MIP (27%), which may be because the sample had such low baseline function (42 ± 14.6 cmH2O). Peak O2, peak cycle power and health-related quality of life also improved significantly compared with the sham group. Pulmonary function, 6-minute walk distance and blood gases remained unchanged. Most recently there has been an uncontrolled study of ‘breathing exercises’ in 22 patients with ankylosing spondylitis. These exercises consisted of diaphragmatic breathing, pursed lip breathing, and thoracic expansion exercises. The patients also used an incentive spirometer equipped with a volume-oriented sustained inhalation (Ortancil et al, 2009). There were significant increases in chest expansion (16%), MIP (9%), MEP (16%) and Bath Ankylosing Spondylitis Functional Index scores after 6 weeks of breathing exercise, but the 6-minute walking distance did not change. The absence of a control group and the unusual nature of the intervention make these data difficult to interpret. The data hint that it may be possible to improve chest wall mobility – which merits further exploration using IMT, which has been shown to increase vital capacity in other conditions. The potential role in improving exertional dyspnoea and exercise tolerance remains unclear as neither study showed a change in 6-minute walking distance. Notwithstanding this, the joint BTS / ACPRC Guidelines make the following recommendation in relation to RMT in patients with kyphoscoliosis: ‘Consider the use of respiratory muscle training’ (Bott et al, 2009).
Finally, it is worthwhile mentioning that repeated, deep inhalations against an inspiratory load have been found to be twice as effective (measured by sputum weight) as standardized physiotherapy consisting of postural drainage and the active cycle of breathing technique (Chatham et al, 2004). Thus, IMT may also facilitate airway clearance.
In summary, interpretation of the evidence relating to the efficacy of RMT in patients with restrictive chest wall disorders is hampered by the very small number of studies. The single placebo-controlled trial suggests that endurance RMT in patients receiving intermittent NPPV may improve peak exercise capacity and health-related quality of life, but not 6-minute walking distance. Further research on strength IMT as a stand-alone intervention is justified, since there is a good theoretical rationale for IMT in patients with restrictive chest wall disorders (see Ch. 3). Furthermore, acute bouts of loaded breathing may also facilitate airway clearance. For specific recommendations regarding implementation of IMT see Chapters 5 and 6.
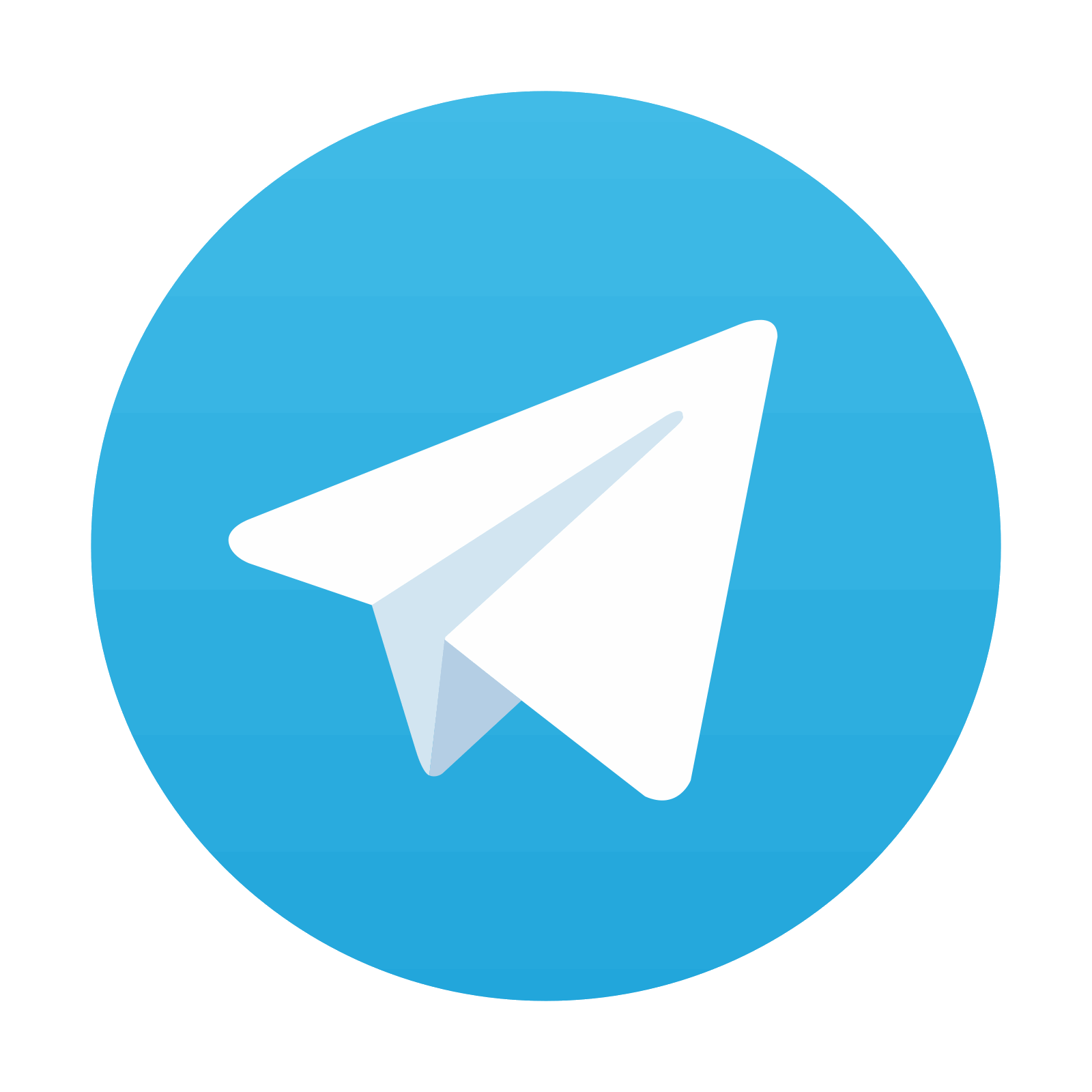
Stay updated, free articles. Join our Telegram channel
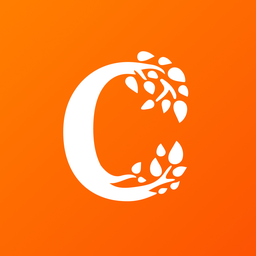
Full access? Get Clinical Tree
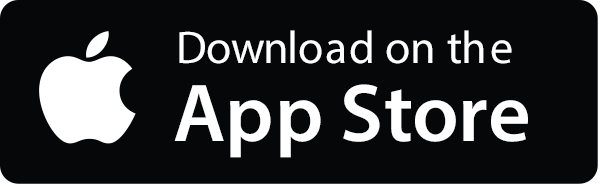
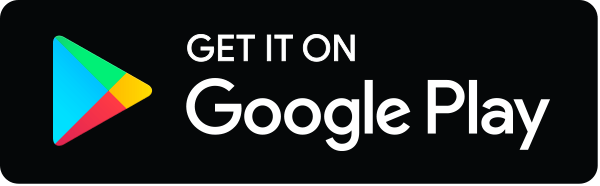