Fig. 19.1
A monolateral external fixator showing progressive destabilization process for enhancing fracture healing, before (a) and after (b) removal of some components of the external fixator
Introduction
Fracture healing and bone repair, an extremely complicated and also a perfect process, have been successfully attracting man’s attention for many years. Although a great number of mechanisms regarding the process have been figured out, much still remains unknown. The healing process starts following an injury. The primary causes of bone injury include trauma, infection, and oncological and metabolic events. In contrast to the healing of soft tissue where injured tissue is replaced by fibrous scar tissue, bone healing occurs through the regeneration of a normal bone tissue. In other words, there is no place for a scar tissue in fracture healing. Moreover, a nonunion occurs as a result of formation of fibrous scar tissue. Thus, fracture healing can be defined as tissue regeneration. In this repair process, there is a cyclical effect created not only by growth factor stimulation but also by the division and differentiation of different cells exposed to inflammation and chemotaxis [1–5].
Fracture repair is closely related to the external factors such as mechanical environment of the fracture site and the type of fixation method. Although most of the factors affecting healing have been revealed in studies, what provides the ideal fracture fixation is still unknown. While the healing process is negatively affected if there is abnormal or gross motion of the fracture ends following reduction or excessive distraction, controlled micro-movement or periodic distraction can accelerate this process [5, 6]. While motion of the fracture site results in healing with the cartilage structure called as endochondral ossification (secondary or indirect healing), on the other hand, absolute lack of movement in the fracture area is characterized by direct bone formation called as intramembranous ossification (primary or direct healing). The vast majority of fractures heal with a combination of these two types of ossifications [4–7]. Endochondral and intramembranous ossifications also occur in the development of long bones. By expanding the bone while growing, the mesenchymal cells in periosteum are differentiated from osteoblasts with intramembranous ossification. In contrast, during the fracture healing and callus distraction, the mesenchymal cells in periosteum are differentiated from chondrocytes, and generally, endochondral ossification occurs [4, 5].
The Histopathological Periods of Fracture Healing
Fracture healing, which is a very complex process, can generally be examined as three overlapping stages. These are the inflammatory, the repair (soft and hard callus), and the remodeling stages. Bone tissue is extremely strong as a structure but fractures occur by excessive forces. When there is a fracture, not only bone tissue is injured, but also the periosteum, muscle tissues, and endosteal and periosteal vascular structures are damaged. The cortex, periosteum, bone marrow, and surrounding soft tissue in the field of injury all contribute to the healing process. A fracture, which is a mechanical event, triggers a reduction in local oxygen and nutrients at the site and secretion of various factors to the area, which will lead to biological events [4, 5].
The inflammatory stage starts with following trauma and the hematoma which is necessary to healing process formed with the cells containing bone marrow of peripheral and intramedullary origin. The major actors in the inflammatory process are cytokines, platelets, bone morphogenetic protein (BMP), and mesenchymal stem cells (MSCs). The inflammatory phase continues for approximately 1 week and progresses with cellular accumulation and macrophages and thrombocytes coming quickly to the injury site. Many growth factors are expressed from the cells. Primarily, these may include platelet-derived growth factor (PDGF), transforming growth factor-β (TGF-β), interleukin-1 and interleukin-6, and cytokines such as prostaglandin E2 [3–5].
The initial pro-inflammatory response includes tumor necrosis factor-α (TNF-α) and interleukin-1 (IL-1), IL-6, IL-11, and IL-18 secretion. These pro-inflammatory cytokines are not only expressed from the macrophages but are also secreted from the MSCs in the periosteum. The secretions peak in the first 24 h after any fracture, and while the levels reduce during the period of cartilage formation, an increase is seen again during the bone remodeling phase [8]. These factors contribute to the accumulation of inflammatory cells and angiogenesis. In addition, cytokines play a role in endochondral bone formation and in the regulation of remodeling.
TNF-α is expressed from the macrophages and the other inflammatory cells, and it is believed that it plays a role in the formation of secondary inflammatory signals and the accumulation of chemotactic factors. IL-1 and IL-6 are the most important interleukins in fracture healing. In addition to the primary cartilaginous callus production of these two interleukin molecules and the angiogenesis-stimulating effects in the injury area, IL-6 also stimulates osteoblast and osteoclast differentiation [3]. All these factors play an active role in starting of the repair process with activation of the cells in the bone marrow, periosteum, and fracture hematoma [3, 5, 9–15].
In the early stage of bony formation, periosteal pre-osteoblasts and local osteoblasts differentiate to new bone with the expression of osteocalcin gene. As mesenchymal cells and fibroblasts proliferate with early new bone formation, they swap with fracture hematoma. The stem cells, which play an effective role in fracture healing, are believed to originate from the bone marrow, periosteum, local muscle, soft tissues, and vascular tissues [4–6, 11]. After maturation of fracture hematoma, a newly formed blood vessel network advances to the healing fracture callus. This vessel network forms a source for the growth factors and progenitor cells which differentiate the mesenchymal cells. The collagenous matrix develops in fracture hematoma and it contains major collagen types. These collagens are Type I and Type II collagens and are responsible for the continuity of the non-cartilaginous and cartilaginous extracellular matrix (ECM), respectively [5, 12]. In the soft callus period, which can last up to 3 weeks, the mesenchymal cells are differentiated from the chondrocytes which will create cartilaginous callus formation. Mature and hypertrophic chondrocytes release Type X collagen and proteases which fragment ECM. At the same time, hypertrophic chondrocytes express transcription factor called as runx2 which regulates ECM proteins such as osteocalcin and osteopontin which are necessary for ossification [4, 5, 12, 16–19].
The differentiation of the hypertrophic cartilage to the bone is noticeable in the advanced stages of fracture healing. This final stage is known as the hard callus period, and soft callus tissue gradually changes to hard callus tissue which will be solid. In this period, the chondrocyte differentiation, apoptosis, ECM fragmentation, revascularization, and bone formation occur [5]. Consequently, cartilage tissue in the medium becomes calcified. At this point, new blood vessels enter the medium, and transformation of the cartilage to bone tissue continues. Finally, matured new bone cannot be distinguished from the original bone, and this period is called as remodeling stage of fracture healing [3–5, 12, 13, 20].
Histo-biological Characteristics of Fracture Healing
For an ideal fracture healing, there are four main biological requirements [5, 9, 14]. Firstly, it is necessary to have a series of the progenitor or MSCs in the right place at the right time. These cells also originate from trabecular bone, surrounding soft tissue including synovia and synovial fluid, the hematopoietic system, bone marrow, and particularly the deep layers of the periosteum, and they play an important role in fracture healing process [5]. Previous studies have supported that osteoblasts and osteocytes, which have a role in the formation of new bone, originate from periosteum, bone marrow, and endosteum, while chondrocytes within the fracture callus primarily originate from the periosteum [5, 15, 16]. Traumatic damage or subtraction of the periosteum leads to the loss of the local source of the multipotent MSCs. Absence of these cells consequences a delay in fracture healing. For bone regeneration, it is necessary to have an accumulation of MSCs that they proliferate and that they differentiate to osteogenic cells. Recent publications have emphasized that accumulation of the circulating systemic MSCs, particularly at the inflammatory response stage, plays an extremely important role in repair process [17–19]. In other words, as a response to the signaling associated with tissue damage, MSCs are sent to the fracture site and participate in the repair process. Primary source of these MSCs originating in the bone marrow is the periosteum cambium layer in fracture healing. Buckwalter et al. demonstrated that, if periosteum is removed, the fracture callus capacity is reduced [20].
The osteoprogenitor cells also produce some molecules such as BMPs, stromal cell-derived factor 1 (SDF-1), and hypoxia-inducible factor-1 α (HIF-α) [3]. These molecules also play an important role in bone repair. While BMP-2 is crucial in bone repair, other BMPs such as BMP-7 are more important in the accumulation of the progenitor cells. In an experimental rat model, the levels of BMP-2, BMP-4, and BMP-7 showed an increase in the early stages of intramembranous ossification [21].
The second requirement for bone repair is the structure of ECM that will create the scaffold to which the progenitor cells can attach. ECM is a dynamic structure and shows continuous changes in order to provide differentiation by gathering together progenitor cells in the bone regeneration process. The main structure of ECM is formed by collagens in cartilage and bone tissues [5]. The main collagen components are Types II, IX, X, and XI for the cartilage matrix and Types I and VI for of the bone matrix. During the bone healing process, ECM keeps on remodeling constantly. This cascade is primarily organized due to the resorption of mineralized cartilage started by macrophage colony-stimulating factor (M-CSF), receptor activator of nuclear factor kappa-B ligand (RANKL), osteoprotegerin, and TNF-α. The most important role of TNF-α is to trigger chondrocyte apoptosis. The absence of TNF-α results in a delayed resorption of mineralized cartilage and prevention of the formation of new bone. Some studies showed that while the level of RANKL is very low in intact bone, it is very high in levels after any fracture [8, 12]. In time, the cartilage tissue is replaced with bone tissue, and this substitution occurs in endochondral ossification; this is one of the most spectacular examples of tissue remodeling [5]. The fracture can gain a more stable structure from soft callus formed from cartilaginous callus in areas of relatively weak mechanical stability. This central hard callus and the bridging which is formed allow weight-bearing on the fracture and ultimately represent a semirigid structure.
The third requirement is some molecular structures which play an effective role in the process of cartilage formation and ossification at different times. Runx2, BMP, TGF-β, and IGF are well-known molecular structures which play a role in osteoblast differentiation [5, 12]. Of these factors, there is a greater understanding of the importance of factor runx2, which plays a direct role in osteoblast differentiation.
The final requirement is to have sufficient blood supply in the region. For successful fracture healing, it is necessary to have a good blood supply and revascularization. Endochondral fracture healing does not remain only within the angiogenesis pathways but also includes cartilaginous degradation to remove the ECM and cells and chondrocyte apoptosis which is necessary for the development of blood vessels at the fracture site [5]. Nowadays, the effect of vascularization of the injured area on repair is a well-known issue. Because of this, it has been attempted to make the surgical methods used for fracture fixation and implant designs appropriate to protect of vascularization in the last few decades [22, 23]. In the endochondral ossification process, the replacement of the hypertrophic cartilage structure with bone tissue requires good vascularization of the environment. The vascular ingrowth within the developing callus tissue is regulated by fibroblast growth factor (FGF), vascular endothelial growth factor (VEGF), and angiopoietins 1 and 2 [3]. The two molecular pathways regulating the vascularization process are the angiopoietin-dependent pathway and the VEGF-dependent pathway [10]. As angiopoietins 1 and 2 are primary vascular morphogenetic proteins, they are expressed in the early stages of the healing cycle. In contrast, VEGF is expressed in the advanced stages of endochondral bone formation. VEGF plays a key role in the regulation of vascular regeneration and is thought to be the most effective factor [3, 24]. VEGF is expressed from both osteoblasts and hypertrophic chondrocytes. Thus, the opportunity is provided for transformation of the avascular cartilaginous matrix to vascularized bone tissue and blood vessel invasion. At the same time, VEGF is an essential mediator in the neo-angiogenesis and revascularization of the fracture site. In addition to VEGF, some other molecules such as TGF, BMP, FGF, and PDGF also play a regulatory role in the vascularization process [4, 5, 24].
Types of Bone Healing
There are two different ways of healing as direct (primary) and indirect (secondary) bone healing [3–5]. Direct healing is achieved by cortical healing cycle after complete anatomic reduction of the ends of fracture. The continuity of the fractured cortexes helps to intersect conic extensions. The bone structure formed without remodeling allows anatomic lamellar bone and rapid Haversian regeneration. If there is any form of movement in the fracture line, indirect fracture healing occurs.
Indirect (Secondary) Fracture Healing
Indirect fracture healing is the most commonly seen form of fracture healing. It can be summarized as a combination of endochondral and intramembranous ossification and subsequent callus formation [3]. This process develops with micro-movements of fracture ends and is accelerated by weight-bearing. The fracture healing which is seen in conservative treatment methods, intramedullary nailing, and external fixation or minimally invasive percutaneous osteosynthesis (MIPO) is called as indirect fracture healing [5, 24] (Fig. 19.2a–c).
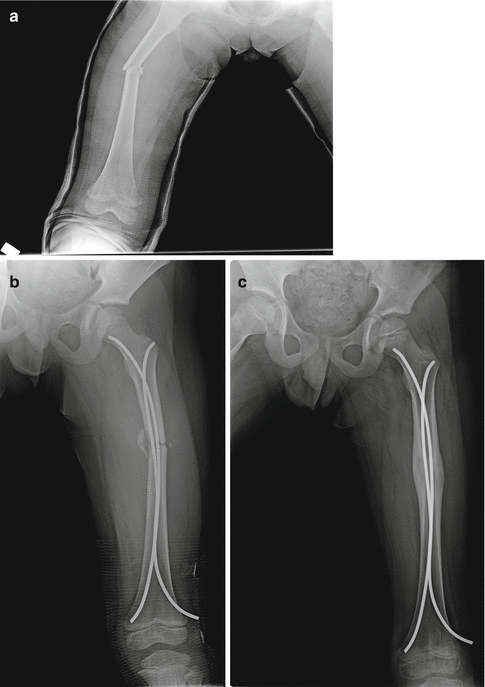
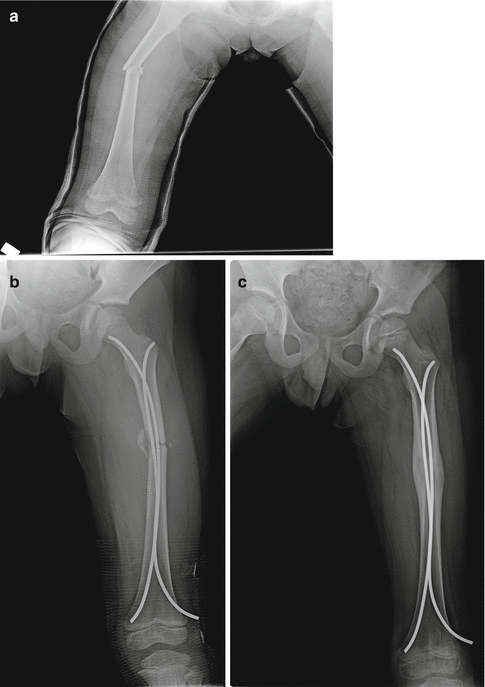
Fig. 19.2
A femoral shaft fracture in an 8-year-old boy (a) was treated by closed intramedullary nailing with elastic nails (b). Note the aberrant callus formation at 6-month follow-up radiograph that suggests indirect bone healing (c)
Indirect fracture healing starts with the collection of fracture hematoma following a fracture. In contrast to the known negative effects of extended and chronically expressed inflammatory cytokines on the bone, joint, and implant materials, after an acute injury, pro-inflammatory molecules expressed short-term and, at a high rate, have a critical and positive effect on tissue regeneration [9]. The acute inflammatory response peaks within 24 h and is completed within 7 days.
Following the inflammatory stage, indirect healing continues with the MSCs and the formation of cartilage callus followed by periosteal callus. Both intramembranous and endochondral ossification occur in indirect bone healing. In time, the cartilage callus is replaced with the mineralized and resorbed bone callus formation. Intramembranous bone formation occurs with osteogenic differentiation of periosteal MSCs without the cartilage stage. Endochondral ossification, however, requires the formation of chondro-progenitor cartilage callus [2].
At advanced stages, the bone regeneration process in a well-vascularized environment requires the transformation of primary soft callus to hard callus by resorption. This stage of fracture healing includes a combination of cell proliferation and differentiation and an increase in the number of cells and matrix deposition [3]. The chondrocytes of the fracture callus become hypertrophic with proliferation, and thus, the ECM becomes calcified. Due to the replacement of woven calcified cartilage with advanced formation of hard callus, the callus becomes stiff and mechanically rigid. Although hard callus provides a rigid structure for biomechanical stability, the biomechanical properties of normal bone cannot be completely regenerated. The secondary resorption stage of the fracture healing cascade starts for a strong bony structure. In this stage, the hard callus is remodeled to lamellar bone with a medullar cavity in the center. While this stage is biochemically regulated by IL-1 and TNF-α is expressed at high rates, it has been observed that the expression of TNF-β is reduced at the same time [3, 5].
The process of remodeling occurs through the balance between resorption of the hard callus by osteoclasts and deposition of the lamellar bone. Although this process starts within 3–4 weeks of the fracture, the complete regeneration of the bone structure continues for years. Axial bone remodeling occurs with stimulation of osteoblastic activity and osteoclastic activity with the formation of electropositivity on the convex side and electronegativity on the concave side, respectively [20]. Thus, step by step, external callus is replaced with lamellar bone. In contrast, the remodeling of internal callus occurs with the formation of the new medullar cavity in the bone diaphysis [1, 3, 9]. Appropriate blood flow and a gradual increase in mechanical stability are indispensable components of successful bone remodeling.
Direct (Primary) Fracture Healing
Direct healing occurs outside of the natural process of fracture healing. For this healing model, anatomic reduction without any space between the fracture ends and rigid fixation are absolute conditions. When these conditions are met, the bone will become lamellar bone over time. Finally, it can be said that from the formation of direct healing, Haversian canals and revascularization are able to be formed. This process can take months or even years.
Direct healing may be in the form of contact healing or gap healing [1–5, 25–27]. In both processes, the structure is reformed by direct anatomic correction and biomechanically sufficient lamellar bone. For direct bone healing, anatomic restoration of fracture fragments is essential. After anatomic placement, the rigid fixation applied significantly reduces the strain between the fracture fragments. Obtaining bone continuity mechanically makes it possible to bring the bone together as a single entity from one cortex to the other [23, 28].
When the gap between the bone ends is <0.01 mm and the strain between the fragments is <2 %, the fracture is defined as contact healing [3] (Fig. 19.3a–c). In these conditions, intersecting conic extensions are formed from osteons in the region closest to the fracture site. By crossing the fracture line, the intersecting conic extensions include osteoclasts, and longitudinal cavities are formed in the bone. These cavities are filled with osteoid tissue formed by osteoblasts. The result is the simultaneous restoration of the Haversian systems and bone union. The newly formed Haversian systems allow for the penetration of blood vessels which carry osteoblastic precursors. The bridging osteons mature by directly remodeling as lamellar bone. Ultimately, fracture healing occurs without the formation of periosteal callus.
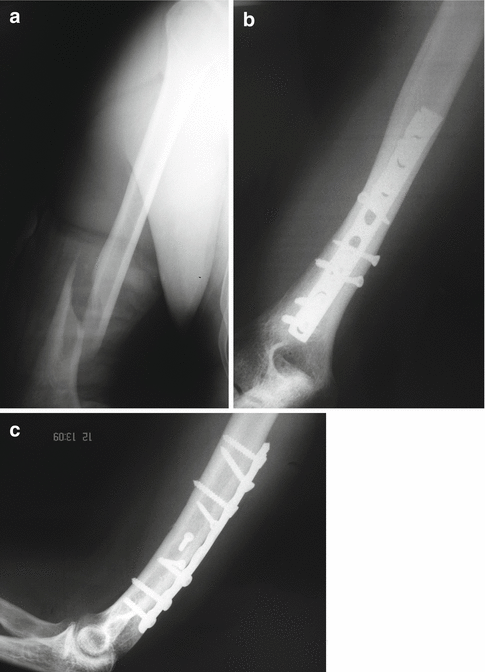
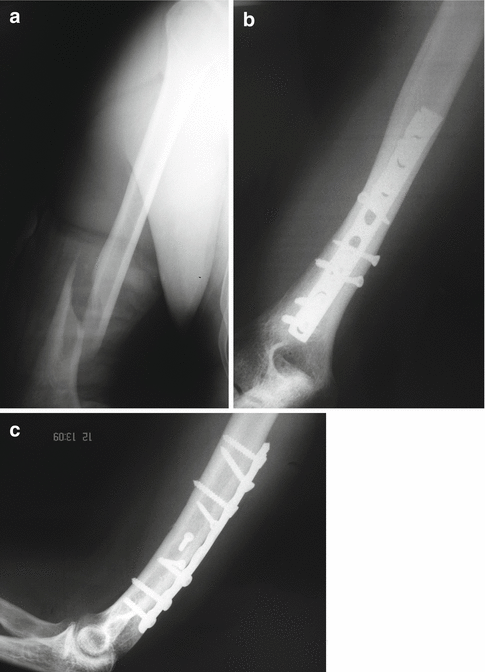
Fig. 19.3
A closed fracture of the distal part of humeral shaft (a) was treated by conventional open reduction and internal fixation with a dynamic compression plate (b). Excellent direct bone healing was achieved after anatomical reduction and rigid fixation of the fracture, and there is no visible callus formation at the last follow-up x-ray (c)
In the second form of direct healing, gap healing, bone union and the remodeling of the Haversian system are different. This situation occurs when there is successful anatomic and stable placement. In this system, the long bone vertical axis is primarily filled with developed lamellar bone. The primary bone structure changes step by step with longitudinal revascularized osteons produced by lamellar bone. This lamellar bone consisted of long vertical axises and it is mechanically weak. The initial process takes 3–8 weeks. Although it is not dense until endochondral remodeling, this stage is necessary for the complete restoration of anatomic and biomechanical properties [26, 28].
Stimulation of Fracture Healing
For many years, scientists have hoped to accelerate fracture healing, and various studies have been conducted related to this. Generally, the methods used to enhance process of the fracture healing can be classified under the two subheadings of biological and physical methods. Biological stimulation is provided with osteoinductive, osteoconductive, or osteogenic factors [1–6, 11, 13, 14, 20, 25, 28]. While the osteoinductive effect has the meaning of stimulating bone formation, the osteoconductive effect is stated for the factors forming an appropriate skeletal frame for fracture healing. The osteogenic effect is stated to mean the factors stimulated for osteogenesis directly from the cells [4, 12, 29]. In addition, the electrical effect and the application of ultrasound can be included in the physical modalities used on the fracture site [2–5, 20, 28].
Autogenous Bone Grafts
Nowadays, autogenous bone grafts are the gold standard graft source [5]. Osteoinductive growth factors are included in osteogenic stem cells which are present in the bone marrow and osteoconductive ECM. Autogenous bone grafts have all these properties together. Osteoinduction occurs through differentiation of MSCs accumulated from the surrounding soft tissue from progenitor cells which make the bone. Osteoconductive materials support osteoprogenitor formation from vascularization as a structural scaffold. Osteogenesis is defined as the process of bone formation. Osteogenic materials, in the context of bone grafting, include live cells such as osteocytes, osteoblasts, and primitive mesenchymal cells which have the capability to differentiate bone. The disadvantages of autogenous bone grafting can include morbidity of the host site, nerve, or artery injuries and infection while harvesting the graft (at rates of 8–30 %) [3–5, 30]. These disadvantages have increased the interest in alternative bone graft sources.
As autogenous cancellous bone grafts have a wider surface area than that of active osteoblasts, the formation of new bone is induced more from cortical bone. Bone formation and resorption occur simultaneously. While osteoclasts resorb dead trabecular bone, osteoblasts express osteoid laydown on the necrotic bone surface. This process is known as “creeping substitution” [5]. As a result, spongious grafts cannot provide immediate structural support to the bone. However, with swift incorporation, the host bone and bone marrow change places within 1 year. With these properties, spongious autogenous bone grafts are an excellent treatment choice in conditions which do not require structural support [3–5].
< div class='tao-gold-member'>
Only gold members can continue reading. Log In or Register a > to continue
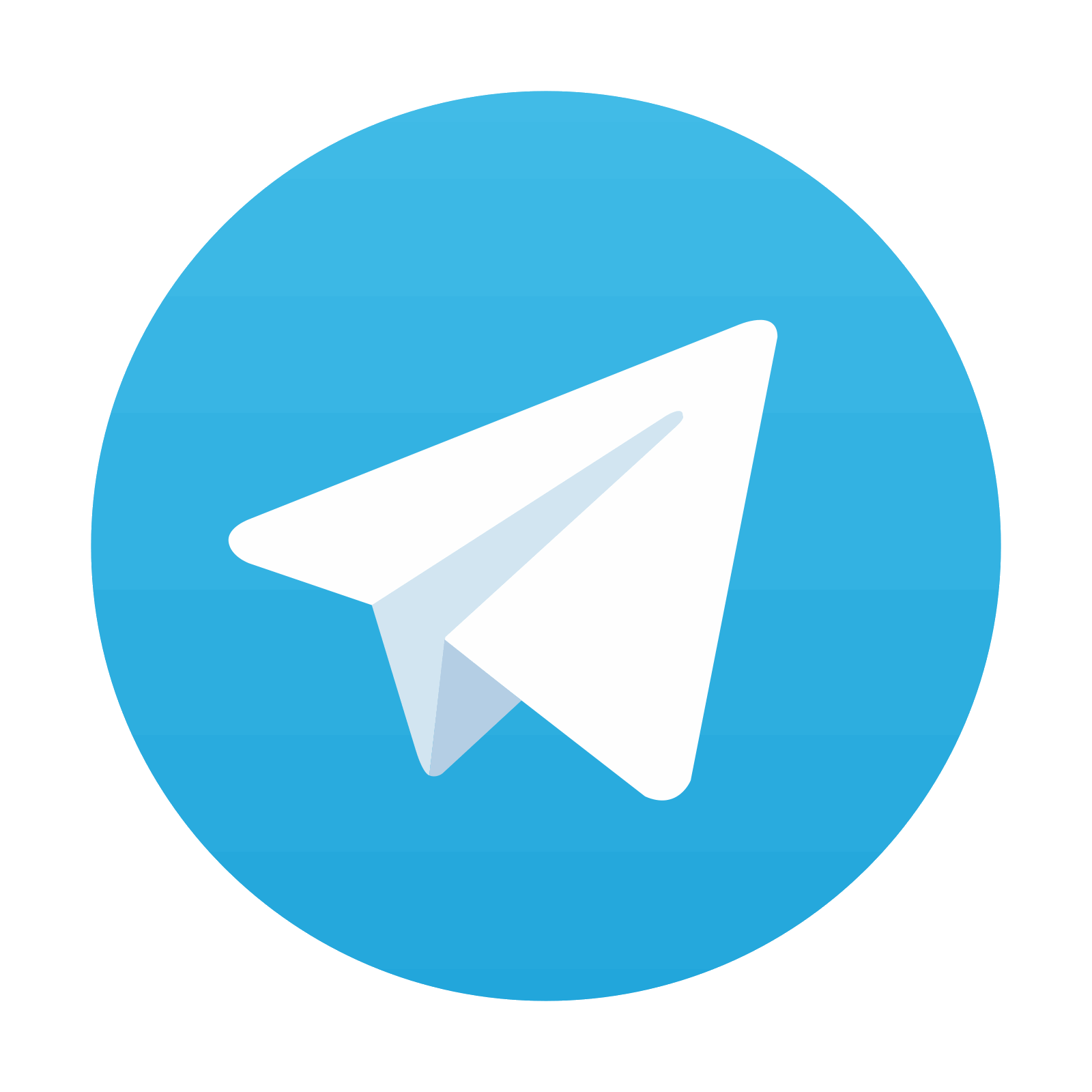
Stay updated, free articles. Join our Telegram channel
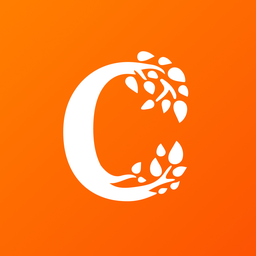
Full access? Get Clinical Tree
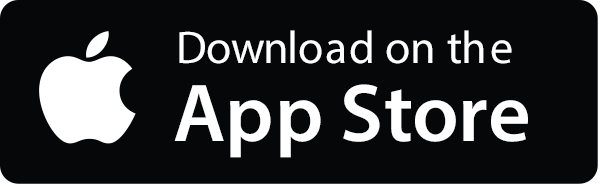
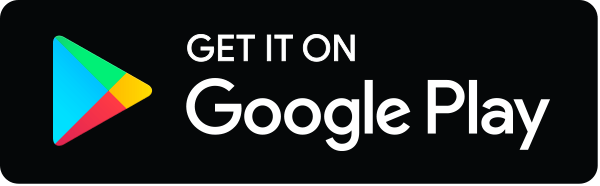