34 Key Points 1. Although many cellular transplantation studies have shown very encouraging results, the application of these approaches to truly repair the chronically injured spinal cord (vs protecting the acutely injured cord) is still a huge challenge. The survival and integration of transplanted cells are still far from optimal and conceivably could be optimized in conjunction with better substrates (e.g., engineered biomaterials and trophic factors). Few preclinical studies have used blunt cervical injuries, and few studies had been performed in the chronic state. 2. The actual mechanisms for how the transplanted cells exert their beneficial effects are poorly understood. Behavioral improvements are likely due to protection, modulation of host plasticity, remyelination of denuded axons, and short-distance axonal regeneration—and these contributions vary among cell types. 3. There is a need for standardization of how to generate and control the quality of cells to minimize variability, especially in autotransplantation settings where the cells are not “off the shelf.” Cell transplantation strategies are extensively investigated for their potential to treat humans with spinal cord injury (SCI). The rationale for studying a particular cell type is based on the availability of the cells as well as the ability of these cells to integrate into the injured spinal cord, where they may myelinate denuded axons or bridge the SCI site and promote axonal sprouting/regeneration. In addition, it has become apparent that many cells used also modify inflammation, provide neuroprotection, and enhance plasticity of the spared host spinal cord, and some cells are doing just that without integration or long-term survival. Given the large number of cell candidates that are reportedly found to be beneficial in animal models of SCI, it is impossible for this chapter to cover the field comprehensively. In addition, the reader has to keep in mind when analyzing preclinical data that even the best-studied cell types can vary considerably among laboratories due to differences in source materials (age, gender, and species from which the cells are taken or progenitors from which the cells are generated), cell purity and contamination with other cell types, culture conditions (such as number of passages), and variability of media used. Hence the focus here is on the cell types best studied in animal models, each followed by a brief outlook on clinical treatment attempts in many parts of the world despite the fact that such interventions have not been validated in controlled clinical trials. Table 34.1 summarizes previous studies in cellular transplantation (Fig. 34.1). Table 34.2 presents the pros and cons of the different cell types. Schwann cells (SCs) are the myelin-forming cells of the peripheral nervous system. Their ability to form cellular bands (of von Buengner) and to support axonal regeneration after nerve injury was recognized almost a century ago.1 This and the ability of SCs to remyelinate central nervous system (CNS) axons sparked the exploration of SCs for the treatment of SCI.2 Over 30 preclinical studies employed either blunt contusion/compression type lesions or full/partial transection injuries of rat spinal cords to treat them with SCs isolated from peripheral nerves of rodents.3 In the sharp injury models, SCs were used in combination with Matrigel (BD Biosciences, Franklin Lakes, NJ) and/or guidance channels providing some scaffold; such models allowed investigation of SCs’ abilities to promote axonal regeneration. There is convincing evidence that SCs enhance the regeneration of sensory axons from the dorsal root ganglia, as well as propriospinal axons adjacent to the injury site.2 However, SCs alone have only limited capacity to promote regeneration of long brain-to-spinal cord projection axons. In any case, those axons regenerating into SC bridges typically fail to reenter the host spinal cord at the other side of the bridge (referred to as off-ramp phenomenon) unless additional treatment strategies are used. In the most prominent study that also achieved behavioral benefits after full spinal cord transections, the SCs were used in combination with a Matrigel-filled polyacrylonitrile/polyvinylchloride channel plus olfactory ensheathing cell (OEC) injections into the stumps plus chondroitinase ABC and mouse immunoglobulin G (IgG).4 Some of the treated rats regained weight-supported stepping, which is arguably the most convincing example of restoration of function after complete spinal cord transection in the preclinical literature. Table 34.2 Pros and Cons of Different Cell Types
Cellular Transplantation in Spinal Cord Injury
Schwann Cells
Schwann cells |
PROS: • Extensively studied cell type that guides axons, remyelinates, and provides neuroprotection (subacute) • Behavioral effects demonstrated by numerous investigators, including efficacy in “chronic” rodent contusion model • Can be harvested from patients for an autologous transplantation approach, which circumvents rejection problems, ethical concerns, and tumor risks |
CONS: • In many cases, appear to require adjuvant treatment to increase efficacy (e.g., Matrigel [BD Biosciences], Rolipram [Tocris Bioscience], cAMP, neurotrophic factors) • Somewhat limited integration into the host compared with neural stem cells • Optimal source for Schwann cells has yet to be determined, and cell quality in autotransplantation setting may be variable |
OECs |
PROS: • Demonstrate good integration into host spinal cord; repeated claims of axonal sprouting and regeneration in partial- or full-transection SCI models, possible myelination and trophic effects • Behavioral improvements have been frequently reported • Offer the possibility of autologous transplantation |
CONS: • No robust behavioral benefits after transplantation into moderate or severe thoracic contusion injuries • Human protocols for OEC cultures still need refinement and cell quality in autotransplantation setting may be variable • In many cases, OECs require cotreatments to increase efficacy (e.g., Schwann cells, Matrigel, Rolipram, cAMP, neurotrophic factors) |
NSPC |
PROS: • Good integration into the host spinal cord • Can differentiate into oligodendroglial cells and myelinate, but likely act by other beneficial mechanisms as well • The majority of studies reported behavioral benefits, including with humans cells |
CONS: • NSPCs do not provide optimal bridges for axonal regeneration • Sourcing of the cells is challenging. Harvesting NSPCs from human fetuses or adult brain material is met with ethical and safety issues and will likely result in variable quality and will require suppression; this difficulty might be overcome by using iPCs |
Human embryonic stem cell–eerived oligodendrocytes |
PROS: • Provide myelin, are neuroprotective, and modify the host endogenous myelination response • Off-the-shelf preparation in production by Geron Pharmaceuticals • FDA-approved phase 1 trials were approved in 2010 |
CONS: • Embryonic stem cell–derived OPCs bear a tumor risk due to possible contamination with pluripotent stem cells • Published preclinical data all from one group; independent replication desirable |
PROS: • Easily harvested for autotransplantations, which circumvents rejection issues • Both human and rodent BMSCs effective in rodent SCI models • Large animal and primate studies successful, as well as studies in chronic contusion injuries (although not independently confirmed) |
CONS: • BMSCs are a somewhat ill-defined populations of cells, hence cell quality hard to assess and likely variable • Integration in the injured spinal cord is limited, mainly neuroprotective and trophic effects • No convincing differentiation into neural cells despite claims to the contrary |
Abbreviations: BMSCs, bone marrow–derived stromal cells; cAMP, cyclic adenosine monophosphate; FDA, US Food and Drug Administration; IPCs, induced pluripotent cells; NSPCs, neural stem/progenitor cells; OECs, olfactory ensheathing cells; OPCs, oligodendrocyte precursor cells; SCI, spinal cord injury.
Fig. 34.1 Cellular transplantation for spinal cord injury. Transplanted cells (green) may remyelinate denuded axons and/or bridge the lesion site (red) where they promote axonal regeneration and/or differentiate into relay neurons that in turn connect onto neurons in the caudal spinal cord (in blue). In addition, transplanted cells may stimulate plasticity in the host spinal cord, e.g., sprouting of spared axons (yellow).
However, the majority of clinical injuries are incomplete and best modeled by blunt contusion injuries. Significant behavioral benefits in open-field locomotion after SC transplantation alone were reported by Takami et al.5 after injecting the SC 7 days after contusion injury and in a study by Barakat et al.6 transplanting into an 8-week chronic contusion injury (the only chronic study in the SC literature). The three other studies saw benefits when SCs were used in combination with either Rolipram (Tocris Bioscience, Minneapolis, MN) plus cyclic adenosine monophosphate (cAMP), or in combinations of methylprednisolone, interleukin (IL)-10, and OECs.7 But even without cotreatments, nerve-derived rodent SCs improved forelimb grip strength and function after transplantation into a cervical contusion injury.8 In light of the fact that a large number of SCIs occur at the cervical level, this is very encouraging.
Human SCs were evaluated in only two studies with thoracic full transections of rats, yielding a small but significant behavioral benefit on the Basso, Beattie, Bresnahan (BBB) scale and the inclined plane test.9 More preclinical experiments with human SCs in cervical and thoracic contusion models are highly desirable. Taken together, the results of SC transplantation into the injured spinal cord show some promise, and the possibility to obtain autologous SCs for transplantation eliminates concerns regarding ethics, contamination, and immune rejection. However, the use of autologous transplants necessitates an additional peripheral nerve injury (e.g., sural nerve excision), which results in additional minor sensory deficits. Recently, alternative sources for SCs from postnatal skin or adult bone marrow have been pursued and tested after thoracic transection10 or contusion11 injuries. Both studies reported modest (but significant) improvements in open-field locomotion, suggesting that other sources that circumvent peripheral nerve biopsies may be an alternative source for autologous SCs. Intriguingly, the SCs from skin-derived progenitors formed bridges across the injury site, migrated into the host parenchyma, and formed myelin with minimal astrocyte hypertrophy.11
Clinical trials with autologous human SCs are moving ahead. Saberi and colleagues12 in Iran recently published the first results from four of 33 patients who underwent autologous transplantation of human SCs into thoracic SCIs of 2 to 6.5 years. No detrimental (nor beneficial) effect of SC transplants was reported in the first four patients; however, magnetic resonance imaging (MRI) failed to detect the SC transplants in the study by Saberi et al.12 This trial, following the International Campaign for Cures of Spinal Cord Injury Paralysis (ICCP) guidelines, is a promising first step in the move toward human clinical trials of SCs for SCI.
Olfactory Ensheathing Cells
OECs are found in the nerve fiber layer of the olfactory bulb and in the nasal olfactory mucosa. OECs have attracted considerable interest because of their ability to facilitate the lifelong growth of olfactory receptor axons from the peripheral nervous system (PNS) environment of the nasal olfactory mucosa to the central nervous system (CNS) environment of the olfactory bulb.13 This sparked the evaluation of OECs in axonal regeneration studies, such as complete thoracic spinal cord transactions, based on the rationale that OECs may promote axonal regeneration across a spinal cord lesion site and facilitate the reentry of axons into the host at the distal host– graft interface, in analogy to the PNS–CNS interface at the olfactory bulb. The study by Ramón-Cueto et al.14 gained great attention due to the apparent regeneration of corticospinal axons and improvements of motor behavior in a grid-climbing test at 3 and 7 months postinjury. When combining this OEC treatment after full transection injury with treadmill step training, the ability of rats to perform plantar stepping was further improved.15 Similarly, in an independent study, Cao et al.16 injected OECs as well as OECs modified to overexpress glial-derived neurotrophic factor into the stumps of the fully transected spinal cord and reported significant motor improvements. However, a study similar to that by Ramón-Cueto et al.,14 this time using primate OECs in nude rats, failed to reveal any corticospinal tract (CST) regeneration and saw only modest regeneration of 5-HT fibers and only transient improvement in behavior.17 Retransection of those animals that demonstrated some improved behavior did not change function, supporting the notion that the improved behavior was not due to long axonal regeneration but to plasticity in the caudal spinal cord.
Studies of axonal regeneration in incomplete transection models were even more equivocal. Although some authors claimed spectacular successes after dorsal column injury or small electrolytic lesions,18 other authors could not confirm axonal growth through and beyond the site of injury.3,19 The reasons for these discrepancies are not fully understood, and sparing of tissue, experimental bias, and variability of the cell culture conditions and sources and animal or injury model systems are likely playing a role.
Human SCIs are typically incomplete and contusive in nature, but none of the studies with OEC transplantation into contusion sites (n = 3) reported that OECs alone conferred any behavioral benefits in either the subacute5 or the chronic setting at 8 weeks postinjury.6 The combination of OECs with SCs, however, seemed to convey significant behavioral benefits, yet in direct comparisons SCs were more effective.5
Only one preclinical study to date has reported benefits with human OECs harvested from the outer layers of the olfactory bulbs from human fetuses of 5 to 7 months’ gestation.20 Although these are p75-positive “OEC-like” cells at best, protocols to harvest OECs from the nasal mucosa of humans are under development to pursue the possibility for autotransplantations. Even in the absence of convincing data with human cells in animals, OECs are already used in some form or another in humans using autotransplantation of mucosal pieces in which OECs are mixed with other cells or in crude cell mixes from the olfactory bulb of aborted fetuses. These reports are uncontrolled and not blinded. Nevertheless, an improvement in 11 of 20 individuals with chronic SCI (> 18 months) and six of 20 persons showing an American Spinal Injury Association Impairment Scale (AIS) score increase from A to C is intriguing,21 although it must not be overlooked that this was in combination with an aggressive physiotherapy regimen. Hence, more basic and clinical studies are needed to understand the biology of human OECs, including these mixed-cell preparations, to improve on the preclinical findings and to design better-controlled clinical trials with better-understood cell preparations.
Neural Stem/Progenitor Cells
Neural stem/progenitor cells (NPCs) are typically harvested from the subventricular zone of the brain or the spinal cord of rodent embryos or adult rodents and amplified as neurospheres in epidermal growth factor (EGF) and/or basic fibroblast growth factor (bFGF) for several rounds of passages. They contain precursors for neurons, astroglia, and oligodendrocytes, and likely some stemlike cells with capacity for self-renewal.
Adult rodent NPCs have been applied to thoracic contusion or compression injuries in rats and mice and to cervical dorsal column transections in rat studies.3 A subacute regimen was chosen in most cases except in the study by Karimi-Abdolrezaee et al.22 Although some authors reported mainly astrocytic differentiation of the transplanted aNPCs,23 many authors observed in addition the expression of up to 60% oligodendroglial markers,3 yet the expression of neuron markers was generally rare (0 to 1%). There is some inconsistency in the extent to which these oligodendrocytes can mature and generate compact myelin in the injured spinal cord.24,25 The majority of the studies using contusion injuries found significant improvements of open-field locomotion scores after transplantation of aNPCs in rats24–26 and in mice.27 However, it needs to be pointed out that cotreatments were used in some of these studies like vaccination with myelin or a cocktail of trophic factors infused intrathecally for 1 week.25 Similarly, only the combination of aNPCs with trophic factors and chondroitinase ABC promoted recovery when administered 8 weeks after a chronic clip compression injury—the first successful chronic treatment with this cell type.22 However, the logistics of obtaining adult NPCs routinely and with consistent quality for treatments of SCI appear difficult; hence embryonic NPCs are also pursued.
Embryonic NPCs (eNPCs) have been assessed in almost a dozen compression/contusion injuries as well as in several full transection or partial transection models of rodents.3 Expression of astrocyte, oligodendrocyte, and neuronal markers was observed to a variable degree. Of interest, eNPC transplantation into cervical weight-compression lesions revealed improvements on a skilled reaching task in rats. In thoracic contusion models, significant effects in open-field locomotion were observed in several studies, and the effects were further enhanced if pretreatments with noggin, which antagonizes bone morphogenic proteins (BMPs), or bFGF-expressing rat amniotic epithelial cells were given. In addition, eNPCs yielded functional benefits in (4/5) sharp lesion models of SCI, including full transections, and the effects where enhanced with various cotreatments.3
Interesting from a translational perspective is the transplantation of eNPCs from human fetuses (8 weeks of gestation) into cervical contusion sites of marmoset monkeys.28 Behaviorally, bar grip power and spontaneous motor activity were improved, which is promising. Given the ethical controversy around the use of human abortion material to harvest human eNPCs as well as the technical variability and logistical problems involved, several authors have pursued human immortalized neural stem cell lines (HB1.F3 clone; line K048 and line c17.23) or long-term human neurosphere cultures29 and transplanted them to rodents. Although some studies were met with behavioral success,29 others reported “pain without gain” with the c17.2 line studied.30 It remains to be seen whether these approaches will yield viable sources of human cells for clinical translation.
A new alternative route for the generation of NPCs was recently opened by the discovery of inducible pluripotent cells from skin fibroblasts31,32 or more recently from peripheral blood.33 As few as four transcription factors (Oct4, Sox2, Klf4, and c-Myc) suffice to convert human skin fibroblast cells into pluripotent cells, which in turn may be induced to differentiate into a wide variety of cells, including NPCs, oligodendrocytes, neurons, or SCs. Hence a patient’s own cells could be used, or more standardized human leukocyte antigen (HLA)-compatible lines could be generated for transplantation into the injured spinal cord. Although the risk of tumors from undifferentiated cells (i.e., teratomas) cannot yet be well assessed, it is hoped that the fast technological advancements in this area will allow for the elimination of undesired pluripotent cells.
Neural and Glial Restricted Precursors, Including Embryonic Stem Cell Derived Oligodendrocyte Precursors
Neural restricted precursors (NRPs) and/or glial restricted precursors (GRPs) are more restricted versions of eNPCs also harvested from embryos. Although transplantation of NRPs alone into uninjured spinal cords resulted in neural differentiation, such neuronal differentiation is far less complete in the environment of the SCI site, underlining that the SCI environment inhibits neuronal differentiation.34 Similarly, GRPs differentiate mainly into astroglial cells in the lesion center, whereas some express oligodendrocyte markers, usually after migration into the spared host spinal cord.35–37 Still, the degree to form myelinating oligodendrocytes by GRPs in the contused spinal cord is somewhat limited, and it appears that behavioral recovery requires the transduction with the neurotrophin D14A38 that has brain-derived neurotrophic factor (BDNF) and neurotrophin-3 (NT-3) activities and also enhances oligodendrocyte differentiation. As with many other transplantation strategies, it can only be speculated to what extent the observed benefits are due to increased myelination, neuroprotection, or neural plasticity.
From a translational perspective, harvesting human GRPs and NRPs from abortion materials is met with logistic and ethical concerns in many countries. Hence alternative sources for oligodendrocyte precursors have been pursued. Most prominent is the differentiation of oligodendrocyte precursor cells (OPCs) from a human embryonic stem cell line, which was recently approved for a phase 1 clinical trial (Geron Inc. www.geron.com/grnopc1 clearance). In essence, these ESC-derived OPCs enhance myelination, are neuroprotective, and mediate moderate improvement of locomotor function when transplanted after acute, but not chronic, SCI.39 This study has not been independently replicated by other laboratories, although Geron performed extensive “in house” safety and efficacy studies on over 2000 rats prior to US Food and Drug Administration (FDA) approval, which has yet to be released to the SCI academic community. Efficacy in blunt cervical models would be desirable if that will be a major human target for translation. Similarly, no larger animal models with OPC transplants exist so far. Concerns regarding the risk of teratoma formation have been voiced due to possible contamination with undifferentiated stem cells.
Bone Marrow–Derived Stromal Cells
Bone marrow cells are a mixture of stromal cells and hematopoietic stem cells. The mesenchymal bone marrow stromal cells (BMSCs) can be isolated and separated from the hematopoietic cell fraction of the bone marrow by their property of adhering to plastic culture dishes. For a systematic review of BMSC transplantation, see Tetzlaff et al.3 BMSCs can differentiate into chondrocytes, osteoblasts, and adipocytes and are thus multipotent precursors; however, the claims of neural differentiation after transplantation into the injured spinal cord have been debated. There is considerable heterogeneity among BMSCs isolated by plastic adhesion, which partly explains the highly variable results among different laboratories regarding the ability of these cells to survive, integrate, and differentiate as neural cells in the injured spinal cord. In addition, there is evidence that rather nonspecific treatments can induce the expression of neuronal markers without truly specifying these cells as neurons or glial cells.40
The majority of the over 20 studies transplanting rodent BMSCs into blunt SCIs (mainly contusions of the rat thoracic spinal cords) reported positive behavioral effects, whereas four failed to see any benefits.3,41–43 In most studies the cells were injected directly into or next to the SCI site, yet, in some hands, intrathecal and even intravenous (IV) delivery is claimed to be successful; although some researchers did not have success with IV delivery. As for most cell transplantations, most studies used a subacute or acute timing for the transplantation, except for Zurita and Vaquero,44 who delayed the treatment to 3 months after spinal cord contusion by weight drop at T6–8. These authors allowed the rats to survive for up to 12 months and claimed significant locomotor improvements. Independent replications are eagerly awaited.
In the light of the widely observed behavioral benefits, it is somewhat surprising that the histological data are very divergent and range from good survival and differentiation of BMSC into neural cells, to poor survival and no differentiation into neural cells. Claims of differentiation are often not credible when in vitro dyes have been used to prelabel the transplanted cells (e.g., the chromatin stain Hoechst17). Still the heterogeneity of histological results once more underlines that beneficial behavioral effects can be brought about by multiple factors, ranging from neuroprotection (via secretion of trophic factors and modification of inflammation) to recruitment of endogenous cells, including stem cells and remyelinating cells and vascular endothelial cells, which enhance endogenous repair, and, last but not least, differentiation and integration as neurons (which is highly debated). Indeed several studies reported more preserved white matter or less cell death, indicative of neuroprotection.3,43 The claims of axonal regeneration in contusion studies can only be interpreted within the site of lesion but not the host spinal cord itself, where spared axons and regenerated axons are not easily distinguishable. Such questions are better addressed in sharp models of SCI, and a few studies with full transections reported mild behavioral benefits.45 Although this may be due to some axonal regeneration, other mechanisms like trophic effects on spinal circuits below the level of the injury site cannot be ruled out. BMSCs do promote axonal growth, and these effects may be attributed to invading SCs (see earlier discussion), and axon growth can be greatly enhanced with co-expression of trophic factors by the transplanted BMSCs.46
Given the fairly easy access to human bone marrow, it does not surprise that almost a dozen studies have been performed with human BMSC, and about two thirds of them reported transplant-related behavioral improvements. Deng and colleagues20 claimed impressive BBB scores of 13 (weight-supported stepping with frequent coordination) versus BBB of 6 in their controls; however, Kim et al.47 found less dramatic benefits (13 vs 10) in a milder contusion model when combining these cells with FGF. Similarly, Cízková et al.48 reported benefits with human BMSC using a balloon compression model. However, Neuhuber et al.49 tested human BMSC from four different donors and found highly variable outcomes in a rat hemisection model using various tests, which underlines the possible heterogeneity of these cells. Hence, it appears that we need a better understanding of the types of cells in the BMSC fraction that are mediating these benefits.
Nevertheless, from a translational perspective, BMSCs are the most widely studied human cells using rodents, large mammals, and primates. This and the easy access to bone marrow for autotransplantation have fueled several unproven human treatment attempts. However, most of these human treatments are using crude mixtures of mesenchymal and hematopoietic cells50–53 and not the cultured plastic-adherent BMSC fractions. Several centers are routinely administering these autologous cell mixes to individuals with chronic SCI, at considerable costs and surgical risks (e.g., www.xcell-center.com). Unfortunately, the assessments are thus far based on small patient cohorts only and are mostly uncontrolled; hence it is difficult to discern any possible BMSC-related benefits from placebo effects or from untethering of the cord and removing scar tissue during surgery. In light of a booming interest in cell transplantation, a systematic preclinical and clinical validation of this approach is overdue.
Other Cells
Several other cell types are claimed to hold promise for the treatment of SCI and could not be dealt with here. These include radial glial cells, amnion epithelial cells, umbilical cord cells (mesenchymal and hematopoietic), adipose-derived mesenchymal cells, hair follicle–derived progenitor cells, skin-derived progenitor cells, and more. Some of these cell types are still not well defined, and there is little independent confirmation regarding their benefits for the injured spinal cord. Nevertheless, uncontrolled clinical treatments with some of these cells are ongoing, as many of these cells are easily obtained without destroying human embryos. More preclinical data are needed before we can judge their true potential for repairing the injured spinal cord.
Outlook
We are seeing very encouraging results with many of the cells discussed in this chapter, yet it is remarkably surprising how few preclinical studies have used blunt cervical injuries (the most frequent clinical injury type), how few studies had been performed in the chronic state, and how little we understand the actual mechanisms whereby the transplanted cells exert their beneficial effects. Behavioral improvements are likely due to protection, modulation of host plasticity, remyelination of denuded axons, and short-distance axonal regeneration—and these contributions vary among cell types. The survival and integration are still far from optimal and conceivably could be optimized in conjunction with better substrates (e.g., engineered biomaterials and trophic factors). In addition, there is the need for standardization of how to generate and control the quality of cells so as to minimize variability, especially in autotransplantation settings where the cells are not “off the shelf.” And, last but not least, the application of these approaches to truly repair the chronically injured spinal cord (vs protecting the acutely injured cord) is still a huge challenge. In the meantime, thousands of patients are traveling around the world to buy unproven cell treatments for their injured spinal cord, reminding us that cell transplantation as such is feasible and that we need to close the gaps in our preclinical data and work effectively toward FDA-approved controlled trials of these promising treatments. The clinical trial by GERON Corporation with human ESC-OPCs was suspended in Fall 2011 due to financial reasons; a clinical safety and feasibility trial of human NSPCs sponsored by STEM CELL INC. was initiated in 2012 in Switzerland.
Pearls
“Stem cells” hold great promise to enhance recovery from SCI by providing cell replacement and thus enhancing multiple mechanisms, including neuroprotection, plasticity in the injured spinal cord, remyelination, and axonal regeneration.
Hence the therapeutic spectrum is broad.
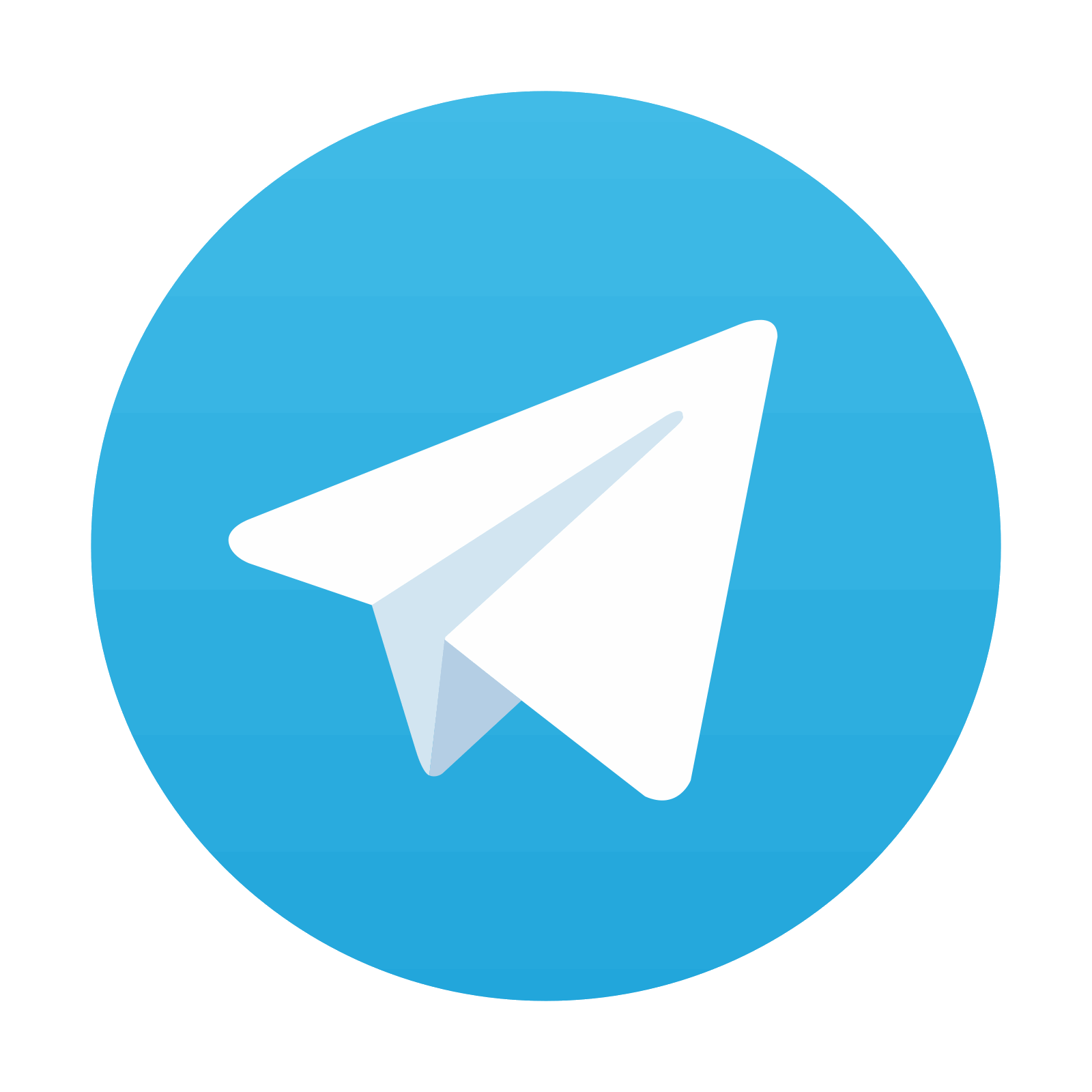
Stay updated, free articles. Join our Telegram channel
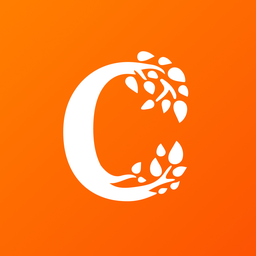
Full access? Get Clinical Tree
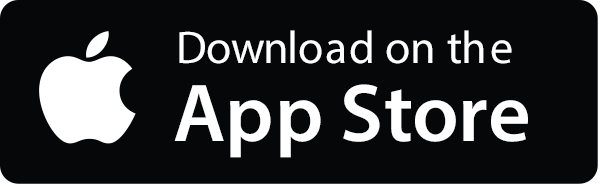
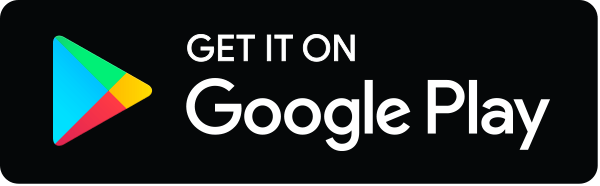