54 Over the last 20 years there have been numerous breakthroughs in the evaluation and management of spinal cord injury (SCI). This chapter discusses ten of the most significant breakthroughs of the last 20 years (Fig. 54.1). The ten advances span seven key areas, with stem cells currently being the most heavily investigated (Fig. 54.2). The ten areas may also be classified into four major themes: repair, neurorestoration, medical management, and surgical management (Fig. 54.3). The ten breakthroughs are not presented in order of importance. Most of the breakthroughs are covered in detail in several chapters in the book, but they are presented here to highlight their importance and to present an overview in a single chapter. The reader is referred to appropriate sections of the book for further details. On average, each mammalian neuron is synaptically connected to 5000 other neurons. Although much attention in recent years has been given to neuroregeneration, including cell replacement and axonal regeneration, the challenges associated with such attempted “rewiring” must not be underestimated. The critical first task in maximizing functional outcome after SCI is preservation of existing circuitry. Fortunately, most SCIs represent contusion rather than transection, and much of the damage is due to secondary cell death, presenting opportunities for meaningful intervention. To this end, enormous gains have been made in the past 20 years in understanding inflammation, excitotoxicity, and free radical effects, as well as apoptotic and neurotrophic pathways (see Chapter 4 for further discussion of pathophysiology). Minocycline is a tetracycline analogue that has been found to mitigate injury in animal models of SCI. Its efficacy is likely due in part to simultaneous disruption of apoptotic pathways via inhibition of mitochondrial cytochrome c, as well as downregulation of multiple inflammatory mediators and microglial activation.1,2 Findings of improved functional outcomes obtained from preclinical studies of SCI in multiple laboratories and multiple species1,3 prompted initiation (in 2004) of a prospective randomized phase 1/2 trial in Calgary (scheduled for completion in 2010). A separate trial employing minocycline in combination with the immunosuppressant tacrolimus is reportedly in progress in Saudi Arabia.3 Riluzole is a sodium channel blocker that additionally inhibits presynaptic calcium channels, thereby blocking glutamate-mediated excitotoxicity after central nervous system (CNS) injury. It has been in use for amyotrophic lateral sclerosis (ALS) since 1997 and was shown in a recent Cochrane meta-analysis to prolong survival by 2 to 3 months.4 Improved recovery of function with riluzole has been observed in animal models of SCI since 1996.3 A phase 1 clinical trial for acute SCI is being undertaken by the North American Clinical Trials Network (NACTN). Plans are under way, based on the outcomes of this study, to undertake a phase 2/3 randomized, controlled trial with riluzole. Fig. 54.1 Ten major breakthroughs in spinal cord Injury research and management in the last 20 years. Clinical trials have also been undertaken with other putative neuroprotective agents, including GM-1, TRH, gacyclidine, nimodipine, and naloxone. However, due to failure to meet primary end points in admittedly often-underpowered clinical studies, these drugs are no longer under active clinical development for SCI.3 Lessons from these trials, including the importance of adequate power and selection of appropriate outcome measures, among other details of trial design, will help to improve the quality of ongoing and upcoming trials. At this time, a surplus of candidate neuroprotective agents, including a variety of multifunctional cytokines, such as VEGF, GDNF, nerve growth factor (NGF), EPO, brain-derived neurotrophic factor (BDNF), and neurotrophin-3 (NT-3), have each shown benefit in animal models of SCI and, together with strategies to promote remyelination, neurite outgrowth, and synaptic plasticity, offer significant hope for the future ability to ameliorate SCI-associated deficits.5–7 As noted elsewhere, multimodal interventions targeting several pathways in the degenerative and regenerative processes may offer the greatest likelihood of achieving functional recovery. Pharmacological inhibition of a single apoptotic pathway, for example, may leave cells vulnerable to death via alternate apoptotic or nonapoptotic pathways. By contrast, interventions to concurrently modulate the inflammatory environment, provide trophic support, enhance free radical scavenging, and promote revascularization may, in combination with a blockade of cell death pathways, yield maximal preservation of neurons and fiber tracts for subsequent remyelination and outgrowth. Fig. 54.2 These breakthroughs span seven main research areas: neuroimaging, neurophysiology, neuromodulation, neuroplasticity, neuroregeneration, neuroprotection, and stem cells, which are currently the center of attention. Fig. 54.3 Ten major breakthroughts, spanning seven main research areas (neuroimaging, neurophysiology, neuromodulation, neuroplasticity, neuroregeneration, neuroprotection, and stem cells, which are the center of attention now) have occurred in SCI research and management in the last twenty years. The breakthroughs can be classified into four thematic areas: repair, neurorestoration, medical management, and surgical management. CNS neurons of certain fish and amphibians are capable of regeneration. However, regeneration of adult mammalian axons is restricted essentially to the peripheral nervous system. Although some limited sprouting of rodent CNS axons was observed by Ramon y Cajal in the 1920s, facilitated by transplants of peripheral nerve,8 it was not until Richardson et al. demonstrated projection of labeled spinal axons across a 10 mm sciatic nerve graft in 19809 that the regenerative capacity of at least some CNS axons was given serious consideration. Thus began a fruitful search for factors that might explain the reluctance of CNS neurons to regenerate in their native environment. Caroni et al. in the late 1980s demonstrated that the myelin membrane of oligodendrocytes is inhibitory to axonal growth.10,11 A monoclonal antibody was developed that could block the action of identified inhibitory fractions from myelin in vitro.12 Administered in vivo, this antibody led to improved functional performance in spinal cord–lesioned rodents.13 Ultimately, the antibody was used to isolate and sequence its target antigen, which was subsequently termed Nogo-A.14 Interestingly, Nogo-A knockout animals showed less consistent regeneration than did wild-type animals treated with Nogo antibodies, suggesting increased inhibition via compensatory pathways.15 A growing list of other myelin-associated factors, components of the glial scar that forms after injury, now exists, each of which has been shown to inhibit neuronal growth cones in vitro. Examples include chondroitin sulfate proteoglycans (CSPGs), a family of inhibitory molecules in the glial scar, myelin-associated glycoprotein (MAG), and oligodendrocyte myelin glycoprotein (OMG).16 Enzymatic digestion of CSPG via chondroitinase ABC has been shown to ameliorate growth cone inhibition, though failure of impaired CSPG synthesis to yield similar results raises the possibility that cleaved glycoaminoglycans (GAGs) may promote neurite outgrowth and underlie the improvements seen with chondroitinase ABC.15 In 2006, Novartis initiated a multicenter open-label phase 1 trial of intrathecal infusion of various doses of ATI355, a humanized Nogo antibody. Results of the anticipated 51 patients were expected in late 2010, but as of this writing they have not been published. Analysis of the signal transduction pathways of growth-cone inhibitory compounds has revealed that all such identified compounds act via activation of the guanosine triphosphatase Rho, which in turn is activated by, and binds to, Rho kinase (ROCK).16 ROCK regulates actin cytoskeleton, and, thereby, growth cone dynamics. McKerracher and Higuchi identified a specific inhibitor of Rho called C3 transferase, produced by Clostridium botulinum, which acts at the final common pathway of growth cone regulation17 and has been shown to promote axonal regeneration and functional recovery in murine SCI.18 Clinical trials have been initiated by BioAxone Therapeutic, with BA-210, a recombinant version of the C3 transferase with improved membrane permeability. This compound (Cethrin, Alseres Pharmaceuticals, Inc., Hopkinton, MA) was administered epidurally within 7 days of injury during spinal stabilization surgery, in combination with fibrin sealant as a delivery vehicle. Results of the open-label phase 1/2a trial, wherein 27% conversion was observed from American Spinal Injury Association (ASIA) grade A to grade B, C, or D, have prompted efforts to translate this into a phase 2/3 randomized, controlled trial.3 The low endogenous levels of adult neurite outgrowth likely represent a mechanism to preserve complex circuitry in higher organisms, thus therapeutic reversal of this stability may open the door for certain unintended effects on sensorimotor function. Moreover, much remains to be learned regarding optimal timing of growth-cone-promoting therapies, as well as the functionality, activity-dependence, and consolidation of any new synaptic connections.19 Nevertheless, ample proof of principle suggests axonal regeneration is likely to be a fundamental contributor to functional regeneration following SCI and may ideally provide critical substrate for neural plasticity, including regulation of central pattern generators (CPGs). Although stem cells have been subject to extraordinarily high expectations, founded more on media hype than on scientific data points, they remain among the most versatile and promising potential therapies for SCI. While neurons from both embryonic and neural stem cells (NSCs) have been shown to survive and form synaptic connections in vivo,20,21 functional benefits in SCI after transplantation of NSCs, MSCs, and other stem or progenitor cells are likely to be attributable in large part to their supportive properties. CNS and bone-marrow-derived stem cells may migrate to the region of injury, modulate inflammation, promote angiogenesis, promote neurite outgrowth, and enhance synaptic plasticity.22 Mechanisms underlying these actions remain incompletely elucidated; however, secretion of a diverse array of neuroprotective compounds has been observed. NSCs are known to exist in the hippocampus and forebrain subventricular zone throughout life. However, numerous groups have additionally reported isolation of NSCs from spinal cord. The exact etiology of such cells is controversial; whereas some argue a latent subventricular zone NSC population exists throughout the neuraxis,23 findings that oligodendrocyte progenitor cells may dedifferentiate into NSCs under in vitro conditions challenge the assumption that NSCs necessarily exist as such in the spinal cord.24 Several attempts have been made to mobilize endogenous stem or progenitor cells via genetic manipulation and infusion of cytokines25; however, a neurogenic response has largely been minimal, and functional consequences may likely be attributable to the treatment itself rather than any new neurons that might be generated as a result.26 Endogenous bone marrow mono-nuclear cells, including macrophages, are mobilized to the site of CNS injury and have been found to be responsible for significant spontaneous recovery.27 Amplification of this response via administration of GCSF28 or even direct transplantation of autologous macrophages has also been shown to yield marked benefit. Transplantation of autologous macrophages at the time of spinal stabilization surgery has been investigated in an open-label phase 1 clinical trial by Procord.29 ASIA score improvement was noted in five of 16 enrolled patients and a phase 2 study was initiated. Benefits from macrophages may be attributable to secretion of prosurvival cytokines and enhanced phagocytosis of potentially harmful injury-associated debris. T cell-based vaccination is based similarly on the principle of timely removal of harmful degradation products as well as provision of growth factors.30 The phase 2 Procord study was halted prematurely due to financial challenges experienced by the sponsoring company. Secondary neuronal death after SCI may be due in part to demyelination. Oligodendrocyte precursor cells (OPCs) from hESCs have been shown to spontaneously remyelinate congenitally myelin-deficient mice. Functional recovery in rats with SCI after hESC-derived OPC transplantation has been robust, and Geron was granted US Food and Drug Administration (FDA) approval for a clinical trial in early 2009.31 The first patients have now been enrolled in the trial. Given the capacity of hESCs to form teratomas, careful attention is appropriately being paid to preclinical safety studies; the trial is currently on hold during investigation of small cysts observed in transplanted animals at later time points. Used in appropriate combinations with other trials, stem cells may have the capacity to mediate significant functional benefits. A recent open-label trial for chronic SCI by Moviglia employed a sequential combination of intraarterial bone marrow mononuclear cells to improve angiogenesis followed by T cell therapy and finally by infusion of autologous NSCs. Although results remain to be replicated by other groups or validated via a randomized double-blind placebo-controlled trial, five of eight treated patients evolved from ASIA A to ASIA D, regaining at least some capacity for ambulation.31 The breakthrough here is the realization that significant plasticity and reorganization occur in the neuraxis after SCI. Transcranial magnetic stimulation and functional magnetic resonance imaging studies have provided evidence of significant plasticity in the sensorimotor cortex after SCI (see Chapter 46 for review). This involves activation of new areas and displacements of activation maxima. This plasticity is dynamic and evolves with time. There is also evidence that significant plasticity accompanies training and rehabilitation.32,33 Clinical implications are unclear at the present time, but the extent and pattern of plasticity may be used as potential markers of recovery. There is significant rewiring of circuits after partial lesions. There is a modest regrowth of axons and a rewiring of circuits so that the cortex can reach the spinal cord through different circuits (via reticulospinal or propriospinal circuits). In that context, the implication of propriospinal pathways has become important.34–36 An area of plasticity research involves attempts to modulate spinal cord plasticity to improve recovery. This area of research is in its infancy and involves modulation of the Hoffmann reflex (H-reflex) and other spinal reflexes, such as reciprocal inhibition, to improve recovery.37–39 Techniques for understanding plasticity and development of new plasticity-enhancing techniques, such as paired association stimulation, prolonged somatosensory stimulation, theta burst stimulation, transcranial direct current stimulation and repetitive transcranial stimulation, represent breakthroughs that are likely to lead to greater enhancement of plasticity and a greater understanding of the relationship between plasticity and recovery.40–48 The breakthrough here was the establishment of the role of the CPG in neurological recovery after SCI. The notion of the CPG is unique and refers to the ability of spinal circuitry to generate an organized rhythmic activity all by itself (of course normally under the control of descending commands and afferents).49 The activity of the CPG is rhythmic, automatic, activated by neurotransmitter or proprioceptive information (e.g., from activity-based training). It can also be activated by pharmacology or electrical stimulation. It is under supraspinal control and can undergo plasticity during training. Significant evidence now exists implicating the CPG in the recovery of locomotion after partial spinal lesions.50,51 There is increasing evidence for a human CPG.52–56 The role of the human CPG in SCI is discussed in detail in Chapter 49. The breakthrough here is the increasing evidence that recovery can be achieved by specific activity-based therapies and the notion that plasticity of spinal circuits is activity dependent. This represents a significant departure from previous rehabilitation philosophies that focused more on development of compensatory strategies. Repetitive practice using task-specific sensory input can engage key spinal locomotor networks and improve recovery.57 Potential mechanisms include increased gene expression, synaptogenesis, increased reorganization, and repair along the neural axis and engagement of the CPG. Physiological benefits include optimization of cardiovascular functioning and musculoskeletal restoration, including increase in bone mineral density, muscle strength, and tone.58 Locomotor training is probably the most well known activity-based therapy and has been shown to increase supraspinal plasticity.32,33 Great interest in human locomotor studies followed the observation that cats with complete transections of the spinal cord recovered locomotor stepping response after intense treadmill training involving partial body weight support and optimization of assistance during stance cycle. Subsequently, several locomotor training regimens have been investigated, including manual and robotic body weight support treadmill training (BWSTT) and functional electrical stimulation augmented ergometry and training methods. The roles in rehabilitation are discussed in detail in the sections on plasticity and rehabilitation (see Chapters 48, 49, and 50). The significant breakthrough here was the realization of the importance of vascular changes in the injured spinal cord with resultant loss of autoregulation and ischemia.59 This basic science knowledge has been translated into the clinical recognition of the importance of blood pressure management in the prevention of secondary injury after acute SCI. Significant evidence has accumulated regarding benefits of close monitoring and aggressive maintenance of blood pressure after significant injury.60–62 An extensive evidence-based guideline recommends avoidance of systolic blood pressure < 90 mmHg or corrected as soon as possible and maintenance of mean arterial pressure at 85 to 90 mmHg during the first 7 days after SCI.63,64 The lack of clinically relevant methods to directly measure spinal cord blood flow remains an ongoing challenge and represents an opportunity for innovation. These are discussed in more detail in Chapter 9. The breakthrough here is the development of imaging technology with the potential to visualize preserved white matter pathways in SCI. This will likely revolutionalize the classification of SCIs based on direct visualization of anatomical tracts. The ASIA scale and motor scores, as well as magnetic resonance imaging (MRI) parameters like the presence of hematoma, have proven valuable in predicting recovery. However, both the ASIA scale and MRI are unable to identify potentially salvageable white matter tracts. This disadvantage will be overcome by diffusion tensor imaging (DTI). DTI also provides quantitative markers of injury, such as the fractional anisotropy and apparent diffusion coefficient, that may become important surrogate markers of recovery (see Chapter 46 for further discussion). Despite major advances in our understanding of neuroregenerative processes, effective regeneration therapy for people with SCI remains elusive. In fact, the improved quality of life, function, and life expectancy after SCI have risen largely from work in specialized SCI medical care and comprehensive rehabilitation. Many medical complications associated with SCI are now preventable or managed more efficiently—thromboembolic disease, pressure ulcers, respiratory insufficiency, male sexual dysfunction, constipation, and renal failure, to name a few. In the absence of a cure for the site of injury, we have sought ways to overcome specific deficits created by SCI—functional electrical stimulation (FES) is the epitome of such endeavors. FES, once merely a sci-fi fantasy, has been enabled by achievements in electronics, microprocessing, neuroscience, and rehabilitation. FES systems now allow certain people with SCI to reclaim meaningful use of their hands, to stand, or to take steps. Some have regained some control of bladder and bowel evacuation,65 others the ability to overcome male sexual dysfunction. Beyond enabling specific motor functions, FES has been shown to increase muscle bulk, improve cardiovascular performance, prevent pressure ulcers, treat osteoporosis and contractures, control spasticity, and improve depression.66,67 The majority of FES systems work by direction stimulation of a peripheral nerve. The energy required to activate muscle contraction via direct muscle fiber stimulation is roughly 100 times that of nerve stimulation—too unsafe to be applied multiple times. The main components of FES systems are basically the same—a power source, command processor, stimulator, lead wires, electrodes, and sensors. Delivery of the stimulation is through a variable number of lead wires to either surface, percutaneous, or implanted electrodes. An external control unit typically houses the power source (batteries), receives information from the user and sensors, and transforms this information into commands that are sent to the stimulator for a specific motor end effect. In open loop control programs, a stimulation results in a specific function (e.g., knee extension) without autocorrection through processing of feedback sensor information. Closed loop programs incorporate information on muscle force and joint motion from sensors and modify the output stimulation accordingly for more complex functions (e.g., taking a step). Surface systems are inexpensive and can be safely placed in the clinic, but accurate placement is difficult, and stimulation often lacks specificity, can be painful, and can be cosmetically unacceptable. Surface systems are best for diagnostics and short-term therapeutic use. Percutaneous leads are often used temporarily for muscle conditioning. They are easier to place accurately than surface electrodes, but long-term use carries the risk of infection and granuloma formation. In implanted FES systems, the electrodes, lead wires, and stimulators are internalized while the control unit and battery are external—they communicate through radio frequency telemetry. Unfortunately, no implantable battery today can meet the energy demand of a multichannel FES system. Naturally, the lower motor neuron (LMN) must be intact for an FES system to work. Other criteria for effective application of FES include the following: (1) the strength of muscle contraction must be forceful, controllable, and repeatable; (2) neural structures must not be damaged by the stimulus; and (3) the method of stimulus delivery must be acceptable to the patient and not be unbearably painful. Several FES systems have been approved by the FDA; a select few are available only in Europe or Japan. Cervical SCI patients with preserved C7 and C8 segments or higher benefit most from upper limb FES systems, given that hand grasp, hold, and release may be restored. Significant spasticity or joint contractures make meaningful use of the hand or arm difficult. Current systems provide some patients the ability to hold and release larger objects like bottles and cans, or even to use finer items like keys. For lower limb function, FES currently enables SCI patients to stand and perform limited transfers, but no system allows for functional walking. In many ways, locomotion is far more complex than it may seem—beyond the loss of large muscles in the lower extremities, most patients with SCI lose proprioceptive feedback as well as control of trunk muscles for maintaining erect posture and balance. Technology limitations include high energy expenditure (due to inadequate efficient coordination of muscle activation), heavy batteries, unreliable hardware, and reliance on visual feedback, among others. FES systems will not replace wheelchairs as the main means of mobility for SCI patients in the foreseeable future. Direct stimulation of the phrenic nerve (i.e., electrophrenic respiration or EPR) either in the cervical or thoracic region allows for some high cervical SCI patients to become independent of ventilatory support.68 Because spontaneous recovery of diaphragm function sometimes occurs even a year after initial injury, people generally wait 4 to 6 months before EPR is considered. The diaphragm often suffers from disuse atrophy, thus commonly 2 to 3 months of diaphragm reconditioning must occur after implantation for complete independence from a ventilator. With adequate teaching, reconditioning, and support, respiratory complications from EPR are equivalent to the current standard and afford patients dramatically improved quality of life. Regular bladder catheterization has dramatically reduced morbidity and mortality from urinary retention in SCI patients. Sacral anterior root stimulation for bladder control has been reported since the 1970s. Refinement of this approach led to development of the Finetech-Brindley bladder system (formerly marketed as “Vocare” in the United States), which has been shown to produce bladder emptying and continence in select SCI patients. The biggest drawback of this technique is the need to perform a dorsal S2-4 rhizotomy, which leads to irreversible loss of reflex erection and ejaculation, to secure continence between stimulation and decrease detrusor and sphincter dyssynergy. Studies show that 80 to 90% of users can urinate on demand, with postvoid residuals of less than 50 mL; between-stimulus continence is preserved in more than 85%. Similar but less well-documented achievements have been made with regard to bowel evacuation. As for male sexual dysfunction, FES of intact anterior sacral nerve roots, especially S2, produces sustained penile erection for the duration of stimulation. Likewise, ejaculation can be generated by stimulation of the presacral sympathetic plexus. However, because other methods of achieving erection and ejaculation exist, FES systems are not typically implanted solely for treatment of male sexual dysfunction. Advances in microelectronics, computer technologies, and neurophysiology have helped create decent FES systems. As components are miniaturized and our understanding of the physiology of myoelectric systems improves, FES systems will surely boast improved functionality and accessibility. With recent research in brain–machine interfaces showing the promise of enabling tetraplegic patients to perform motor tasks by thought, we can be optimistic about the future for SCI patients69 (see Chapters 52 and 54 for further discussion). For decades, the prevailing attitude about SCI was that all the damage to the CNS occurs at the time of injury and that the damage is irreversible. Hemodynamic stabilization of the trauma patient claimed top priority. Because outcomes were thought to be totally determined by the extent of initial injury, little attention was paid to examining the effect of prompt interventions, such as drugs or decompressive surgery, on the “natural course” of SCI. It is now recognized that much of the degeneration of the spinal cord after SCI is due to a multifactorial secondary injury process that occurs over minutes, to hours, to days. For instance, production of reactive oxygen-induced lipid peroxidation (LP) has been found to play a key role in this process. Demonstration of LP inhibition by the glucocortico steroid methylprednisolone (MP) in animal SCI models led to important clinical trials in humans, the results of which have been the focus of intense debate for nearly 20 years.70–74 However, due to concerns regarding the relatively small effect sizes seen in the National Acute Spinal Cord Injury Study 2 (NASCIS-2) and NASCIS-3 trials, coupled with potential adverse effects of immunosuppression on wound healing and resistance to infection, many clinicians have questioned whether steroids should be routinely used in acute traumatic SCI. Currently, the use of steroids is not considered standard of care by several evidence-based guidelines.70,75–78 To clarify, the guidelines committee of the AANS/CNS Joint Section on Disorders of the Spine and Peripheral Nerves concluded that use of methylprednisolone in treatment of acute SCI in adults can only be supported as a “treatment option” and not a standard of care.75 The controversy surrounding the use of steroids is discussed in detail in Chapter 11. Early surgical decompression for acute SCI is another area of controversy where recent research seems to be pushing practice paradigms in a new direction. Decompression of the injured spinal cord is thought to prevent or attenuate secondary injury mechanisms. This theory is supported by work done in purebred dogs,79,80 where a time-dependent benefit in hindleg motor function from early surgical decompression done within 6 hours of injury was observed. Rabinowitz et al.79 further showed that this effect was independent of administration of methylprednisolone. Despite the widespread use of surgery in patients with SCI, its application varies widely. The presence and duration of a therapeutic window were merely suggested by numerous Class III and limited Class II studies.81–83 The Surgical Treatment for Acute Spinal Cord Injury Study (STASCIS) group was formed in 1992 by the Spinal Cord Injury Committee of the Joint Section on Neurotrauma and Critical Care of the AANS and CNS, with the goal of conducting prospective, controlled trials to determine the role and timing of decompressive surgery after acute SCI.74,84 Of the 222 patients with follow-up available at 6 months postinjury, 19.8% of patients undergoing early surgery showed a 2 grade improvement in AIS, compared to 8.8% in the late decompression group (OR = 2.57, 95% Cl:1.11,5.97). In the multivariate analysis, adjusted for preoperative neurological status and steroid administration, the odds of at least a 2 grade AIS improvement were 2.8 times higher amongst those who underwent early surgery as compared to those who underwent late surgery (OR = 2.83, 95% Cl:1.10,7.28). They concluded that “Decompression prior to 24 hours after SCI can be performed safely and is associated with improved neurologic outcome, defined as at least a 2 grade AIS improvement at 6 months follow-up.”85 It is important to note that the STASCIS study enrolled only patients with subaxial cervical spine injury. Upon conclusion of the study and publication of the results, careful analysis must be done before any new treatment guidelines are made, but positive results may shift current practice paradigms toward more urgent management of spinal cord compression (see Chapter 27 for further discussion). It is noteworthy that a consensus panel of the Spine Trauma Study Group has recommended early intervention for patients with traumatic SCI and that this approach is favored by the vast majority of clinicians, as determined by a recently published international survey.86,87 1. Teng YD, Choi H, Onario RC, et al. Minocycline inhibits contusion-triggered mitochondrial cytochrome c release and mitigates functional deficits after spinal cord injury. Proc Natl Acad Sci USA 2004;101(9):3071–3076 PubMed 2. Wells JE, Hurlbert RJ, Fehlings MG, Yong VW. Neuroprotection by minocycline facilitates significant recovery from spinal cord injury in mice. Brain 2003;126(Pt 7):1628–1637 PubMed 3. Hawryluk GW, Rowland J, Kwon BK, Fehlings MG. Protection and repair of the injured spinal cord: a review of completed, ongoing, and planned clinical trials for acute spinal cord injury. Neurosurg Focus 2008;25(5):E14 PubMed 4. Miller RG, Mitchell JD, Lyon M, Moore DH. Riluzole for amyotrophic lateral sclerosis (ALS)/motor neuron disease (MND). Cochrane Database Syst Rev 2007; (1):CD001447 PubMed 5. Lu P, Tuszynski MH. Growth factors and combinatorial therapies for CNS regeneration. Exp Neurol 2008;209(2):313–320 PubMed 6. Onose G, Anghelescu A, Muresanu DF, et al. A review of published reports on neuroprotection in spinal cord injury. Spinal Cord 2009;47(10): 716–726 PubMed 7. White RE, Jakeman LB. Don’t fence me in: harnessing the beneficial roles of astrocytes for spinal cord repair. Restor Neurol Neurosci 2008;26(2-3): 197–214 PubMed 8. Ramon y Cajal S. Degeneration and Regeneration of the Nervous System. Vol 2. Haffner Publishing; 1928 9. Richardson PM, McGuinness UM, Aguayo AJ. Axons from CNS neurons regenerate into PNS grafts. Nature 1980;284(5753):264–265 PubMed 10. Caroni P, Savio T, Schwab ME. Central nervous system regeneration: oligodendrocytes and myelin as non-permissive substrates for neurite growth. Prog Brain Res 1988;78:363–370 PubMed 11. Caroni P, Schwab ME. Two membrane protein fractions from rat central myelin with inhibitory properties for neurite growth and fibroblast spreading. J Cell Biol 1988;106(4):1281–1288 PubMed 12. Caroni P, Schwab ME. Antibody against myelin-associated inhibitor of neurite growth neutralizes nonpermissive substrate properties of CNS white matter. Neuron 1988;1(1):85–96 PubMed 13. Bregman BS, Kunkel-Bagden E, Schnell L, Dai HN, Gao D, Schwab ME. Recovery from spinal cord injury mediated by antibodies to neurite growth inhibitors. Nature 1995;378(6556): 498–501 PubMed 14. Chen MS, Huber AB, van der Haar ME, et al. Nogo-A is a myelin-associated neurite outgrowth inhibitor and an antigen for monoclonal antibody IN-1. Nature 2000;403(6768):434–439 PubMed 15. Rolls A, Shechter R, Schwartz M. The bright side of the glial scar in CNS repair. Nat Rev Neurosci 2009;10(3):235–241 PubMed 16. Yiu G, He Z. Glial inhibition of CNS axon regeneration. Nat Rev Neurosci 2006;7(8):617–627 PubMed 17. McKerracher L, Higuchi H. Targeting Rho to stimulate repair after spinal cord injury. J Neurotrauma 2006;23(3-4):309–317 PubMed 18. Dergham P, Ellezam B, Essagian C, Avedissian H, Lubell WD, McKerracher L. Rho signaling pathway targeted to promote spinal cord repair. J Neurosci 2002;22(15):6570–6577 PubMed 19. Maier IC, Ichiyama RM, Courtine G, et al. Differential effects of anti-Nogo-A antibody treatment and treadmill training in rats with incomplete spinal cord injury. Brain 2009;132(Pt 6): 1426–1440 PubMed 20. Cizkova D, Kakinohana O, Kucharova K, et al. Functional recovery in rats with ischemic paraplegia after spinal grafting of human spinal stem cells. Neuroscience 2007;147(2):546–560 PubMed 21. Yan J, Xu L, Welsh AM, et al. Extensive neuronal differentiation of human neural stem cell grafts in adult rat spinal cord. PLoS Med 2007;4(2): e39 PubMed 22. Xu XM, Onifer SM. Transplantation-mediated strategies to promote axonal regeneration following spinal cord injury. Respir Physiol Neurobiol 2009;169(2):171–182 PubMed 23. Horner PJ, Power AE, Kempermann G, et al. Proliferation and differentiation of progenitor cells throughout the intact adult rat spinal cord. J Neurosci 2000;20(6):2218–2228 PubMed 24. Kondo T, Raff M. Chromatin remodeling and histone modification in the conversion of oligodendrocyte precursors to neural stem cells. Genes Dev 2004;18(23):2963–2972 PubMed 25. Carlén M, Meletis K, Barnabé-Heider F, Frisén J. Genetic visualization of neurogenesis. Exp Cell Res 2006;312(15):2851–2859 PubMed 26. Ohori Y, Yamamoto S, Nagao M, et al. Growth factor treatment and genetic manipulation stimulate neurogenesis and oligodendrogenesis by endogenous neural progenitors in the injured adult spinal cord. J Neurosci 2006;26(46):11948–11960 PubMed 27. Shechter R, London A, Varol C, et al. Infiltrating blood-derived macrophages are vital cells playing an anti-inflammatory role in recovery from spinal cord injury in mice. PLoS Med 2009;6(7):e1000113 PubMed 28. Luo J, Zhang HT, Jiang XD, Xue S, Ke YQ. Combination of bone marrow stromal cell transplantation with mobilization by granulocyte-colony stimulating factor promotes functional recovery after spinal cord transection. Acta Neurochir (Wien) 2009;151(11):1483–1492 PubMed 29. Knoller N, Auerbach G, Fulga V, et al. Clinical experience using incubated autologous macrophages as a treatment for complete spinal cord injury: phase I study results. J Neurosurg Spine 2005;3(3):173–181 PubMed 30. Ziv Y, Avidan H, Pluchino S, Martino G, Schwartz M. Synergy between immune cells and adult neural stem/progenitor cells promotes functional recovery from spinal cord injury. Proc Natl Acad Sci U S A 2006;103(35):13174–13179 PubMed 31. Moviglia GA, Varela G, Brizuela JA, et al. Case report on the clinical results of a combined cellular therapy for chronic spinal cord injured patients. Spinal Cord 2009;47(6):499–503 PubMed 32. Thomas SL, Gorassini MA. Increases in corticospinal tract function by treadmill training after incomplete spinal cord injury. J Neurophysiol 2005;94(4):2844–2855 PubMed 33. Winchester P, McColl R, Querry R, et al. Changes in supraspinal activation patterns following robotic locomotor therapy in motor-incomplete spinal cord injury. Neurorehabil Neural Repair 2005;19(4):313–324 PubMed 34. Zaporozhets E, Cowley KC, Schmidt BJ. Propriospinal neurons contribute to bulbospinal transmission of the locomotor command signal in the neonatal rat spinal cord. J Physiol 2006;572(Pt 2):443–458 PubMed 35. Courtine G, Song B, Roy RR, et al. Recovery of supraspinal control of stepping via indirect propriospinal relay connections after spinal cord injury. Nat Med 2008;14(1):69–74 PubMed 36. Cowley KC, Zaporozhets E, Schmidt BJ. Propriospinal neurons are sufficient for bulbospinal transmission of the locomotor command signal in the neonatal rat spinal cord. J Physiol 2008;586(6): 1623–1635 PubMed 37. Perez MA, Field-Fote EC, Floeter MK. Patterned sensory stimulation induces plasticity in reciprocal Ia inhibition in humans. J Neurosci 2003;23(6):2014–2018 PubMed 38. Thompson AK, Chen XY, Wolpaw JR. Acquisition of a simple motor skill: task-dependent adaptation plus long-term change in the human soleus H-reflex. J Neurosci 2009;29(18):5784–5792 PubMed 39. Wolpaw JR. Spinal cord plasticity in acquisition and maintenance of motor skills. Acta Physiol (Oxf) 2007;189(2):155–169 PubMed 40. Beekhuizen KS, Field-Fote EC. Massed practice versus massed practice with stimulation: effects on upper extremity function and cortical plasticity in individuals with incomplete cervical spinal cord injury. Neurorehabil Neural Repair 2005;19(1):33–45 PubMed 41. Belci M, Catley M, Husain M, Frankel HL, Davey NJ. Magnetic brain stimulation can improve clinical outcome in incomplete spinal cord injured patients. Spinal Cord 2004;42(7):417–419 PubMed 42. Fregni F, Boggio PS, Lima MC, et al. A sham-controlled, phase II trial of transcranial direct current stimulation for the treatment of central pain in traumatic spinal cord injury. Pain 2006;122(1-2):197–209 PubMed 43. Fregni F, Pascual-Leone A. Technology insight: noninvasive brain stimulation in neurology-perspectives on the therapeutic potential of rTMS and tDCS. Nat Clin Pract Neurol 2007;3(7):383–393 PubMed 44. Huang YZ, Edwards MJ, Rounis E, Bhatia KP, Roth-well JC. Theta burst stimulation of the human motor cortex. Neuron 2005;45(2):201–206 PubMed 45. Stefan K, Kunesch E, Cohen LG, Benecke R, Classen J. Induction of plasticity in the human motor cortex by paired associative stimulation. Brain 2000;123(Pt 3):572–584 PubMed 46. Talelli P, Greenwood RJ, Rothwell JC. Exploring Theta Burst Stimulation as an intervention to improve motor recovery in chronic stroke. Clin Neurophysiol 2007;118(2):333–342 PubMed 47. Valero-Cabré A, Pascual-Leone A. Impact of TMS on the primary motor cortex and associated spinal systems. IEEE Eng Med Biol Mag 2005;24(1):29–35 PubMed 48. Valle AC, Dionisio K, Pitskel NB, et al. Low and high frequency repetitive transcranial magnetic stimulation for the treatment of spasticity. Dev Med Child Neurol 2007;49(7):534–538 PubMed 49. Grillner S, McClellan A, Sigvardt K, Wallén P. On the spinal generation of locomotion, with particular reference to a simple vertebrate: the lamprey. Birth Defects Orig Artic Ser 1983;19(4):347–356 PubMed 50. Barrière G, Leblond H, Provencher J, Rossignol S. Prominent role of the spinal central pattern generator in the recovery of locomotion after partial spinal cord injuries. J Neurosci 2008;28(15): 3976–3987 PubMed 51. Rossignol S, Barrière G, Alluin O, Frigon A. Re-expression of locomotor function after partial spinal cord injury. Physiology (Bethesda) 2009; 24:127–139 PubMed 52. Nadeau S, Jacquemin G, Fournier C, Lamarre Y, Rossignol S. Spontaneous motor rhythms of the back and legs in a patient with a complete spinal cord transection. Neurorehabil Neural Repair 2010;24(4):377–383 PubMed 53. Minassian K, Persy I, Rattay F, Pinter MM, Kern H, Dimitrijevic MR. Human lumbar cord circuitries can be activated by extrinsic tonic input to generate locomotor-like activity. Hum Mov Sci 2007;26(2):275–295 PubMed 54. Dietz V. Spinal cord pattern generators for locomotion. Clin Neurophysiol 2003;114(8): 1379–1389 PubMed 55. Dimitrijevic MR, Gerasimenko Y, Pinter MM. Evidence for a spinal central pattern generator in humans. Ann N Y Acad Sci 1998;860:360–376 PubMed 56. Calancie B. Spinal myoclonus after spinal cord injury. J Spinal Cord Med 2006;29(4):413–424 PubMed 57. Behrman AL, Nair PM, Bowden MG, et al. Locomotor training restores walking in a nonambulatory child with chronic, severe, incomplete cervical spinal cord injury. Phys Ther 2008;88(5):580–590 PubMed 58. Sadowsky CL, McDonald JW. Activity-based restorative therapies: concepts and applications in spinal cord injury-related neurorehabilitation. Dev Disabil Res Rev 2009;15(2):112–116 PubMed 59. Tator CH, Fehlings MG. Review of the secondary injury theory of acute spinal cord trauma with emphasis on vascular mechanisms. J Neurosurg 1991;75(1):15–26 PubMed 60. Vale FL, Burns J, Jackson AB, Hadley MN. Combined medical and surgical treatment after acute spinal cord injury: results of a prospective pilot study to assess the merits of aggressive medical resuscitation and blood pressure management. J Neurosurg 1997;87(2):239–246 PubMed 61. Ploumis A, Yadlapalli N, Fehlings MG, Kwon BK, Vaccaro AR. A systematic review of the evidence supporting a role for vasopressor support in acute SCI. Spinal Cord 2010;48(5):356–362 PubMed 62. Ahn H, Fehlings MG. Prevention, identification, and treatment of perioperative spinal cord injury. Neurosurg Focus 2008;25(5):E15 PubMed 63. Management of acute central cervical spinal cord injuries. Neurosurgery 2002;50(3, Suppl): S166–S172 PubMed 64. Blood pressure management after acute spinal cord injury. Neurosurgery 2002;50(3, Suppl): S58–S62 PubMed 65. Creasey GH, Grill JH, Korsten M, et al; Implanted Neuroprosthesis Research Group. An implantable neuroprosthesis for restoring bladder and bowel control to patients with spinal cord injuries: a multicenter trial. Arch Phys Med Rehabil 2001;82(11):1512–1519 PubMed 66. Hamid S, Hayek R. Role of electrical stimulation for rehabilitation and regeneration after spinal cord injury: an overview. Eur Spine J 2008;17(9):1256–1269 PubMed 67. Ragnarsson KT. Functional electrical stimulation after spinal cord injury: current use, therapeutic effects and future directions. Spinal Cord 2008;46(4):255–274 PubMed 68. Glenn WW, Brouillette RT, Dentz B, et al. Fundamental considerations in pacing of the diaphragm for chronic ventilatory insufficiency: a multi-center study. Pacing Clin Electrophysiol 1988;11(11 Pt 2):2121–2127 PubMed 69. Hochberg LR, Serruya MD, Friehs GM, et al. Neuronal ensemble control of prosthetic devices by a human with tetraplegia. Nature 2006;442(7099):164–171 PubMed 70. Fehlings MG; Spine Focus Panel. Summary statement: the use of methylprednisolone in acute spinal cord injury. Spine (Phila Pa 1976) 2001;26(24, Suppl):S55 PubMed 71. Bracken MB, Holford TR. Neurological and functional status 1 year after acute spinal cord injury: estimates of functional recovery in National Acute Spinal Cord Injury Study II from results modeled in National Acute Spinal Cord Injury Study III. J Neurosurg 2002; 96(3, Suppl)259–266 PubMed 72. Bracken MB, Shepard MJ, Collins WF Jr, et al. Methylprednisolone or naloxone treatment after acute spinal cord injury: 1-year follow-up data: results of the Second National Acute Spinal Cord Injury Study. J Neurosurg 1992;76(1):23–31 PubMed 73. Bracken MB, Shepard MJ, Collins WF, et al. A randomized, controlled trial of methylprednisolone or naloxone in the treatment of acute spinal-cord injury: results of the Second National Acute Spinal Cord Injury Study. N Engl J Med 1990;322(20):1405–1411 PubMed 74. Baptiste DC, Fehlings MG. Update on the treatment of spinal cord injury. Prog Brain Res 2007;161:217–233 PubMed 75. Pharmacological therapy after acute cervical spinal cord injury. Neurosurgery 2002;50(3, Suppl):S63–S72 PubMed 76. Hugenholtz H. Methylprednisolone for acute spinal cord injury: not a standard of care. CMAJ 2003;168(9):1145–1146 PubMed 77. Hurlbert RJ. Methylprednisolone for acute spinal cord injury: an inappropriate standard of care. J Neurosurg 2000;93(1, Suppl):1–7 PubMed 78. Sayer FT, Kronvall E, Nilsson OG. Methylprednisolone treatment in acute spinal cord injury: the myth challenged through a structured analysis of published literature. Spine J 2006;6(3):335–343 PubMed 79. Rabinowitz RS, Eck JC, Harper CM Jr, et al. Urgent surgical decompression compared to methylprednisolone for the treatment of acute spinal cord injury: a randomized prospective study in beagle dogs. Spine (Phila Pa 1976) 2008;33(21): 2260–2268 PubMed 80. Delamarter RB, Sherman J, Carr JB. Pathophysiology of spinal cord injury: recovery after immediate and delayed decompression. J Bone Joint Surg Am 1995;77(7):1042–1049 PubMed 81. Fehlings MG, Perrin RG. The role and timing of early decompression for cervical spinal cord injury: update with a review of recent clinical evidence. Injury 2005;36(Suppl 2):B13–B26 PubMed 82. Fehlings MG, Perrin RG. The timing of surgical intervention in the treatment of spinal cord injury: a systematic review of recent clinical evidence. Spine (Phila Pa 1976) 2006;31(11, Suppl):S28– S35, discussion S36 PubMed 83. Fehlings MG, Tator CH. An evidence-based review of decompressive surgery in acute spinal cord injury: rationale, indications, and timing based on experimental and clinical studies. J Neurosurg 1999;91(1, Suppl):1–11 PubMed 84. Ng WP, Fehlings MG, Cuddy B, et al. Surgical Treatment for Acute Spinal Cord Injury Study pilot study #2: evaluation of protocol for decompressive surgery within 8 hours of injury. Neurosurg Focus 1999;6(1):e3 PubMed 86. Fehlings MG, Wilson JR. Timing of surgical intervention in spinal trauma: what does the evidence indicate? Spine (Phila Pa 1976) 2010;35(21, Suppl):S159–S160 PubMed 87. Fehlings MG, Rabin D, Sears W, Cadotte DW, Aarabi B. Current practice in the timing of surgical intervention in spinal cord injury. Spine (Phila Pa 1976) 2010;35(21, Suppl):S166–S173 PubMed
Breakthroughs of the Last Twenty Years
Neuroprotection
Axonal Regeneration
Stem Cell Therapy and Cellular Transplantation
Plasticity
Central Pattern Generator
Activity-Based Restorative Therapy
Management of Blood Pressure
Development of Diffusion Tensor Imaging
Functional Electrical Stimulation
Early Interventions after Spinal Cord Injury: Steroids and Surgical Decompression in Acute Spinal Cord Injury
References
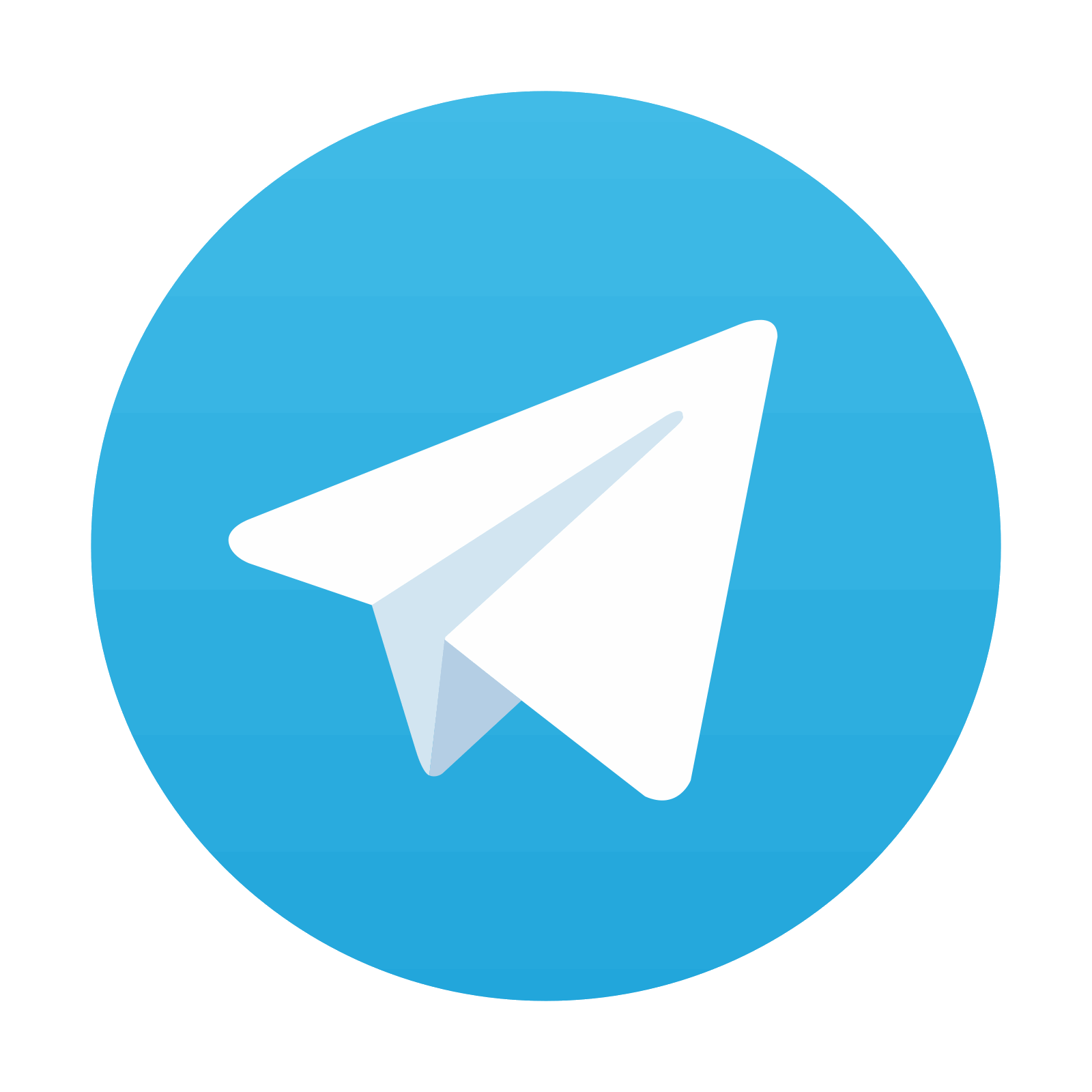
Stay updated, free articles. Join our Telegram channel
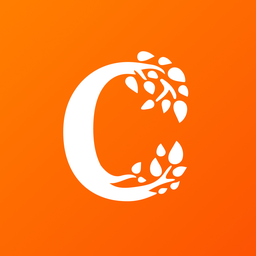
Full access? Get Clinical Tree
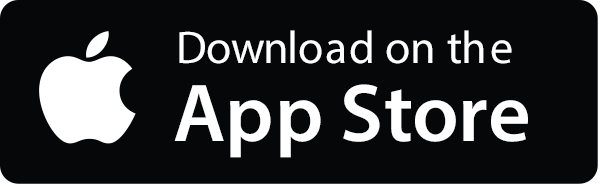
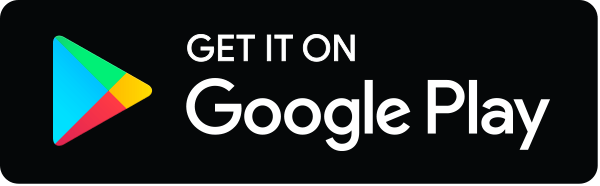