Biomechanics of the Natural Hip Joint
Drew N. Stal, Stephen Ferguson, Stephen J. Incavo and Philip C. Noble
Key Points
Introduction
The hip joint plays a significant role in the human osteoarticular system, both in terms of locomotion and as a load-bearing joint for the torso by transmitting weight to different areas of the pelvis.1,2 In an effort to improve the diagnosis and treatment of various pathologic and structural abnormalities of the hip, it is essential to acquire a basic understanding of hip biomechanics. This includes the anatomy of the hip joint, its normal range of motion (ROM), and the function of the hip musculature during gait.
Advances in this field have included the development of more effective methods of evaluating joint function and understanding pathologic conditions, the scientific investigation of alternative surgical approaches for hip reconstruction, and the development of methods for measuring joint forces and moments developed in vivo. In addition, the application of biomechanical principles has helped shed new light on dysmorphic conditions compromising normal hip motion, whether they stem from acquired abnormalities (e.g., post-traumatic deformities, Perthes disease, slipped capital femoral epiphysis [SCFE]), developmental abnormalities (e.g., congenital dysplasia of the hip [CDH], developmental dysplasia of the hip [DDH]), or abnormalities of unknown origin (e.g. cam deformity of the femoral head–neck junction, and pincer deformities of the acetabular margin). Ongoing investigations of the biomechanics of the capsule, labrum, and femoroacetabular impingement (FAI) hold the promise of revealing the underlying mechanism of “idiopathic” coxarthrosis.
Kinematics of the Normal and Diseased Hip
Any review of the biomechanics of the hip should address both the kinematics and the kinetics of normal hip function. Joint kinematics is the study of angular or translational motion of the hip in response to applied forces. Kinetics is the study of forces and moments acting on the joint, most commonly during stance, gait, or functional activities. Typically, these forces are created by the balance between gravity, acting to pull the body to the ground, and muscle contraction, which serves to keep the skeleton aloft. This balance relies on the transmission of load by intermediate structures such as tendons, ligaments, the hip capsule, and the articular tissues.
The study of hip biomechanics can be approached in several ways. Motion analysis can be used to quantify joint kinematics, especially in correlation with analytical models of the musculoskeletal system. Consequently, joint force calculations can be made using data obtained from gait and force platform measurements, in conjunction with analytical models that simulate the force of contraction and the line of action of the corresponding musculature.
The hip joint is classified as an enarthrodial ball and socket joint that allows for polyaxial articulation between the body and the lower extremity. The femoral head comprises nearly two-thirds of a sphere, whereas the mating acetabulum forms a hemisphere of the same diameter. The cartilaginous surfaces of the femur and the acetabulum are not perfectly conforming, in that the femoral head corresponds more to a conchoid than a sphere.3 This permits the hip joint to undergo movement in an assortment of motion axes that allow flexion-extension, abduction-adduction, and internal-external rotation. Despite a sturdy articular capsule and ligamentous stability, the hip joint allows a great deal of mobility of the femur with respect to the pelvis. Joint motion is greatest in the sagittal plane, with the femur flexing and extending around a left-right axis.4 With the knee flexed, the hip can be actively flexed to approximately 120 degrees before further motion if limited by the joint capsule; flexion of the hip with the knee fully extended is limited to only 90 degrees because of hamstring tension.2,4,5 Passive hip flexion reaches 140 degrees when the knee is flexed.6 Overall, existing data for maximum ROM values include 120 degrees for flexion, 20 degrees for extension, 45 degrees for abduction, 30 degrees for adduction, and 40 degrees for internal and external rotation.6,7
Gender/Racial Differences
In discussing normal hip kinematics, it is important to note ROM variations due to age, gender, ethnicity, and geographic location. It is difficult to establish an all-encompassing set of hip ROM values because of the subjectivity of a given population. By and large, studies have shown that females walk with higher cadence and with a shorter stride length, as well as with similar comfortable ambulatory rates, compared with men.8,9 These findings were corroborated in a study by Kerrigan and associates,10 who found females to exhibit a statistically significant increase in peak hip flexion but a decrease in hip extension compared with male subjects, yet overall kinematic and kinetic joint patterns were similar between genders.10 In a comprehensive retrostudy by the First National Health and Nutrition Examination Survey, as reported by Roach and colleagues,11 hip ROM measurements were recorded from a sample of 1683 subjects broken down by age (25-39, 40-59, and 60-74 years), sex, and ethnicity (white and African American).11 Upon comparison of age alone, hip flexion, extension, abduction, and internal-external rotation all decreased with age, but not significantly. Additional measurements are shown in Table 1-1.
Table 1-1
Difference in Mean Active Range of Motion (in Degrees) for Ages 25–39 Years Compared With Ages 60–74 Years by Sex and Race Groups
From Roach KM: Normal hip and knee active range of motion: the relationship to age. Phys Ther 71:656–664, 1991.
A majority of normal hip ROM investigation involves subjects from the Western hemisphere. This has led to accepted values for hip ROM, yet these values cannot necessarily pertain to subjects from non-Western cultures, who participate in different ADLs. In many Middle Eastern and Asian countries, ADLs involve postures that necessitate a larger range of flexion at the hip, knee, and ankle joints.12 For instance, cross-legged sitting posture is popular in Asia during dining, as is kneeling during prayer in the Middle East and Japan, and can be maintained easily for hours.12 Activities such as stretching, kneeling, and gardening are more common in North America.12 In a study by Ahlberg and co-workers,13 joint ROM was measured among 50 Saudi Arabian males, who exhibited greater hip flexion, abduction, and external rotation compared with normative data (130.8 degrees, 50.8 degrees, and 72.9 degrees, respectively), and less hip extension, adduction, and internal rotation (13.9 degrees, 30.1 degrees, 36.7 degrees, respectively).13 This increase can be correlated with differences in repeated habitual activities involving squatting and kneeling compared with Western cultures. Similar findings were reported by Hoaglund and associates while examining Chinese subjects versus white subjects in Hong Kong, China.14
Because of geographic variation in ADLs such as squatting, kneeling, and sitting cross-legged, many studies have measured hip ROM in non-Western cultures in these corresponding positions to accompany normal ROM values. In a study of the range-of-motion of the joints of nonwestern subjects conducted by Mulholland and colleagues,15 hip flexion reached 130 degrees during full squat and 90 to 100 degrees while sitting cross-legged; hip external rotation ranged from 5 to 36 degrees for full squat and from 35 to 60 degrees while sitting cross-legged, and hip abduction ranged from 10 to 30 degrees for full squat and from 40 to 45 degrees while sitting cross-legged.15 Measurements of ROM of the hip during these activities are reported in Table 1-2 for a group of Indian subjects.12
Table 1-2
Range of Motion of the Hip During Functional Activities*
*All values are expressed as the average ± standard deviation; units are degrees.
From Hemmerich A, et al: Hip, knee, and ankle kinematics of high range of motion activities of daily living. J Orthop Res 24:770–781, 2006.
Structures Controlling Hip Motion
To understand the basic kinematics of the hip joint, it is instructive to review in detail the basic anatomy of passive stabilizers of the hip, including the capsular ligaments, the acetabular labrum, and the ligamentum teres.
The Hip Capsule and Ligaments
During abduction and adduction, limb movement occurs around an anteroposterior axis, as well as in the frontal plane. Average hip abduction has been estimated at 50 degrees.4 The hip capsule (capsular ligament) is critical to the stability of the joint during abduction and adduction and serves as a constraint in preventing dislocation at the extremes of motion.16,17
The capsule is a complex structure formed by three discrete ligaments: iliofemoral, femoral arcuate (pubofemoral), and ischiofemoral ligaments. The anteriorly located iliofemoral ligament, the largest and one of the strongest ligaments of the hip joint, serves to restrict extension and limit internal rotation. The ligament itself consists of two bands extending from the anterior-inferior iliac spine medially to two insertion sites along the intertrochanteric line laterally. The femoral arcuate ligament is located anteromedially and is attached to the superior ramus of the pubis; it connects to the femoral neck, helping to limit abduction and external rotation. Last, the ischiofemoral ligament is situated posteriorly and runs horizontally across the posterior surface of the femoral neck from the acetabular rim and the labrum to the inner surface of the greater trochanter. It serves to limit internal rotation and adduction during hip flexion. Several studies have demonstrated through mechanical testing that the ischiofemoral ligament is the weakest of the capsular ligaments,17 which makes the joint susceptible to posterior dislocation.18
The Acetabular Labrum
The acetabular labrum, a fibrocartilaginous lip attached to the bony margin of the acetabulum, deepens the acetabular socket, substantially extending coverage of the femoral head. The labrum is characterized by a three-layer structure, with the inner layer at the articular surface covered by a fine mesh of type II collagen fibrils, below which one finds a lamellar collagen structure and finally an outer periphery composed of dense connective tissue with fibers oriented circumferentially. In an extensive histomorphologic study, Won and co-workers19 identified several key features at the anterior portion of the labrum, which other studies have reported as the predominant area for labral tears, including a tall triangular shape with apex heights of up to 7 mm and sublabral clefts, perpendicular to the articular surface, at the interface between the labrum and the acetabular rim. The labrum is an avascular tissue with only limited blood supply in the peripheral third of the tissue from the adjacent capsule.20,21 Mechanical properties of the labrum are highly anisotropic, with preferential stiffness in the circumferential direction22 and strong dependence of its mechanical competence on gender, anatomic location, and the degenerative state of the hip.23,24 Labral tears were first cited as a potential source of hip pain by Altenberg more than 30 years ago.25 Labral tears may result from trauma, hypolaxity of the capsule, dysplasia, or impingement.
Although a link between labral pathology and joint degeneration has been proposed, only recently has the biomechanical function of the labrum been well understood. In the normal hip joint, the labrum contributes very little in the way of direct mechanical resistance to joint loading, despite its position and prominence at the acetabular rim.26 However, the compliant and elastic labrum serves as a mechanical seal around the periphery of the joint, enhancing lubrication by effectively blocking passage of synovial fluid into and out of the joint.27–29 This sealing property is readily demonstrated by the well-known suction effect observed during distraction or dislocation of the hip in surgery and has been proven to increase joint stability and to distribute compressive loads in a more uniform manner, thereby decreasing surrounding cartilage stress30–32 (Figs. 1-1 and 1-2). In a series of computer simulations and in vitro experiments,29,31,33 we have shown that the labrum allows a layer of synovial fluid to be maintained between the femur and the acetabulum, thus preventing direct contact of the articulating surfaces during short-term loading. With this sealing effect, loads are transferred across the joint predominantly by uniform pressurization of interstitial fluid of the cartilage layers, not by direct solid-on-solid contact stresses. In the absence of this seal, deformation of the solid matrix of the cartilage is substantially greater. However, in vitro experiments have shown that both sealing mechanisms are highly dependent on the fit of the compliant labrum against the femoral head.31
Figure 1-1 Average load required to distract the femur a distance of 1 mm, 3 mm, and 5 mm with the labrum intact, vented to release the partial vacuum, and incised to simulate a full-thickness tear. (Redrawn from Crawford MJ, et al: The 2007 Frank Stinchfield Award: the biomechanics of the hip labrum and the stability of the hip. Clin Orthop Relat Res 465:16–22, 2007.)
Figure 1-2 Predicted distribution of cartilage contact stresses at (A) 1000 seconds, and (B) 10,000 seconds after load application with the labrum (dark gray) and without the labrum (light gray). (Redrawn from Ferguson SJ, et al: The influence of the acetabular labrum on hip joint cartilage consolidation: a poroelastic finite element model. J Biomech 33:953–960, 2000.)
It has been proposed that the labrum may enhance retention of a boundary layer of lubricant even after fluid film depletion.34 Over long-term loading (i.e., the diurnal cycle), the labrum contributes an important source of resistance to the flow of interstitial fluid that is expressed from the cartilage layers of the joint under load. Cartilage layer consolidation, in principle similar to the wringing out a sponge, is up to 40% faster following labral excision. This, in turn, has a dramatic influence on internal stresses within the cartilage layers, as the center of pressure is shifted toward the acetabular rim, and subsurface shear strains are increased at the subchondral interface, which may contribute to delamination.29
Damage to the labrum through injury or pathology can compromise its sealing function, resulting in subtle but critical destabilization of the hip (Fig. 1-3). This may lead to a shift in the center of rotation of the joint, thereby increasing acetabular rim loading and potentially hastening the onset of early osteoarthritis (OA) and joint disease caused by sustained cartilage erosion.27,28,35,36 Despite early descriptions of the labrum as a continuous structure connecting to the articular cartilage throughout the acetabulum, studies have shown that the anterior aspect makes minimal contact with the acetabular cartilage compared with the posterior aspect.27,37 Consequently, tearing of the acetabular labrum will occur predominantly anteriorly as the result of inferior mechanical properties leading to hip instability, as well as watershed labral lesions, which ultimately can lead to degenerative joint disease.27,28,35,36
Figure 1-3 Change in displacement of the femoral head within the acetabulum when loaded with an abduction moment in 20 degrees of external rotation for hip joints tested with the labrum intact, then vented, then torn. (Redrawn from Crawford MJ, Dy CJ, Alexander JW, et al: The biomechanics of the hip labrum and the stability of the hip. Clin Orthop Relat Res 465:16–22, 2007.)
Reattachment of the damaged labrum has been suggested to partially restore its original function. This procedure is intended to avoid compromising the biomechanical function of the labrum caused by surgical débridement, which may otherwise lead to degenerative changes associated with OA. Although the long-term results of labral reattachment are still unknown, short-term follow-up is positive,38 with both improved clinical results and less prevalent signs of joint degeneration.39 A comprehensive labral repair using the ligamentum teres capitis has been proposed as a further step toward restoration of normal joint function.40
The Ligamentum Teres
The ligamentum teres is an intra-articular ligament that attaches the acetabulum and the femoral head. Specifically, two bands connect to the ischial and pubic sides of the acetabular notch in congruence with the transverse ligament of the acetabulum, and insert into the fovea capitis femoris.41,42 To date, a paucity of investigative studies have explored the exact function of the ligamentum teres and its role in stability of the hip. It has been postulated that the ligamentum teres plays a role in stability, but corroborative data are scarce. It is known that the structure is taut during hip adduction, flexion, and external rotation—positions in which the joint is least stable—which demonstrates its potential role in hip stability.43 A biomechanical study of immature porcine ligaments conducted by Wenger and colleagues42 showed that ligaments followed a stepwise stress-strain curve.42 The mode of failure was discovered to be ligamentous disruption from the acetabulum, then avulsion from the femoral head with signs of mid- or intraligamentous tears.41,42 Additional clinical and biomechanical studies of adult ligamentum teres are needed to conclusively determine the nature of its role in hip stability.
Hip Joint Motion During Normal Gait
The form of the hip joint permits motion of the lower extremity under the control of specific muscles. Researchers are particularly interested in examining the motion of the natural hip joint in basic locomotion. Gait analysis is also convenient because this process of motor development is fully integrated in hip patients, which simplifies comparison of gait parameters between individuals.4
During gait activities, the hip joint plays a crucial role in advancement of the lower extremity. Typically, one gait cycle commences when the heel of the reference limb makes contact with the ground, and it concludes when the toe of the same limb leaves the ground (i.e., measuring one full stride length of the reference limb).2,4,44 The gait cycle is divided into two phases: stance (60% gait cycle) and swing (40% gait cycle).2,4 Stance phase is subdivided into initial contact, loading response, midswing, terminal stance, and preswing. During stance phase, the body is propelled forward while being supported by the limb touching the ground. Because the supporting limb is ahead of the body at heel-strike (i.e., the hip is flexed) and is behind the body at toe-off (i.e., the hip is extended), the center of gravity of the body moves in an epicyclical pattern. Swing phase is subdivided into initial swing, midswing, and terminal swing, and occurs when the supporting limb is lifted free of the ground and is driven forward ahead of the body in preparation for the next cycle of weight-bearing support. During this phase, an open-chain loading configuration is present, as the foot is not constrained through ground contact, so that the extremity can rotate freely.
In a standard stride in the sagittal plane, the hip traverses two arcs of motion: flexion to extension during stance phase, and extension to flexion during swing phase. During each gait cycle, the average arc of motion of the hip is 40 degrees in the sagittal plane, ranging from 30 degrees of flexion to 10 degrees of hyperextension with respect to the neutral standing position (zero flexion).2,45 At initial limb contact, the thigh is already in 20 degrees of flexion and is relatively stable.2 Hip adduction reaches a maximum of 5 degrees in the loading response of the late stance phase.4 The hip gradually extends as the limb approaches mid stance (10% to 30% gait cycle). Continuing at the same ambulatory rate, the thigh reaches the zero position at 38% of the gait cycle.2 The femur then enters preswing (50% to 60% gait cycle), aligning in a posterior position with peak hip extension at 10 degrees.2,4 The hip reaches a maximum abduction angle of 5 to 7 degrees as medial rotation occurs at the closing stages of the loading response.4
At the end of the stance period (60% gait cycle), the hip enters flexion and reaches neutral hip position, although the thigh is still in several degrees of extension.2 The hip attains maximum flexion of 30 to 35 degrees at approximately 85% of the gait cycle in the terminal swing phase as the hip rotates laterally,2,4,46 before returning to the beginning of the gait cycle. In the coronal plane, a small amount of adduction and abduction of the hip occurs as the non–weight-bearing portion of the pelvis moves through the gait cycle. During stance, the hip is initially 10 degrees adducted; as the load increases, the hip shifts to 5 degrees adduction until terminal stance is attained. At the onset of the swing phase, the hip abducts minimally at around 5 degrees.2 In the transverse plane, the hip exhibits both internal and external rotation throughout the gait cycle, with peak internal rotation occurring at the conclusion of the loading response, and maximum external rotation at the launch of the swing phase (end of preswing). The net transverse internal-external hip motion is 8 degrees, with total thigh rotation of around 15 degrees.2
The Role of Muscles in Hip Motion
Extension
The hip extensor muscles, primarily the hamstrings, adductor magnus, and gluteus maximus, operate from the late midswing phase through the loading response. At the end of the midswing phase, the semimembranosus, semitendinosus, and long biceps femoris all simultaneously begin gradual contraction before peaking in early terminal swing. Semirelaxation of these muscles ensues before complete relaxation at the end of the swing phase. The adductor magnus initiates contraction at the end of the terminal swing, which increases during initial contact; it remains active during the loading response before tapering off. The lower half of the gluteus maximus contracts at the end of the terminal swing and increases its strength of contraction through heel-strike until the end of the loading response. Contraction of the gluteus maximus acts as a brake in slowing down the momentum of the lower limb in the terminal swing phase, in preparation for the stance phase. Because the action of body weight is to extend the hip joint, the gluteus maximus plays a critical role in enabling individuals to walk up an incline or a set of stairs, and to run and jump.47
Abduction
Hip abduction is more pronounced during the first half of the stance phase and involves the action of the gluteus medius, tensor fascia lata, and upper gluteus maximus. By acting medially, these muscles compensate for the contralateral drop of the pelvis caused by the force of overall body weight. The gluteus medius and the upper gluteus maximus initiate contraction at the end of terminal swing (95% gait cycle); this peaks after initial contact and then continues throughout midstance. Variation in the activity of the tensor fascia lata muscle is noted, as the posterior portion of the muscle contributes most during the loading response, while the anterior portion is only active during terminal stance.
Flexion and Adduction
The flexor muscles play a critical role in the function of the hip in normal gait and are active from late terminal stance to early in midswing as the hip is elevated free of the ground and carried through. Specific muscles contributing to this function are the adductor longus, rectus femoris, gracilis, pectineus, tensor fasciae latae, iliopsoas, sartorius, and iliacus muscles. The chief flexor is the iliopsoas, which stems from the ventral surface of the transverse processes and the sides of the lumbar vertebrae and connects to the iliac portion, inserting immediately below the lesser trochanter.47 Key muscles in the anterior aspect of adduction include the pectineus, adductor magnus, longus, brevis, and gracilis; the posterior aspect includes the gluteus maximus, quadratus femoris, obturator externus, and hamstrings.
Pelvic Motion
The pelvis acts similar to a stationary unit with coordinated motion occurring between the lumbar spine and the hip joint as the result of muscle coordination. Pelvic motion at the hip consists of anteroposterior pelvic tilting, lateral pelvic tilting, and pelvic rotation. Anterior pelvic tilting occurs via the hip flexors and trunk extensors as the result of contraction of the iliopsoas, which pulls the pelvis anteriorly and inferiorly; the extensors of the lumbar spine pull the pelvis superiorly.47 This causes inferior movement of the symphysis pubis, increasing the lordotic curve of the lumbar spine. An opposite event is the decrease in the lordotic curve of the lumbar spine, which results in posterior pelvic tilt; this brings the posterior aspect of the pelvis closer to the posterior surface of the femur, resulting in hip extension as the hip extensor muscles work with the trunk flexors (rectus and obliquus muscles).47 Lateral flexion and rotation of the vertebral spine cause lateral pelvic tilting, resulting in hip adduction or abduction.
Joint Motion, Gait, and Functional Adaptations
As individuals pursue more demanding ADLs, it is reasonable to predict that any limitations in hip function and range of motion will tend to compromise each individual’s expectations and physical activities. Whether as the result of pathologies such as premature wear and tear of the hip joint and degenerative arthritis or artificial joint replacement, individuals will ultimately alter their normal functional movements to compensate for joint pain, muscle weakness, or instability. The Trendelenburg gait pattern, for example, occurs when patients experience a decrease in function of their abductor muscles as a result of reduced muscle strength or abductor moment arm length.48 This leads to a compensatory gait pattern to reduce the demand placed on the abductor muscles by moving the trunk closer to the affected hip. Femoroacetabular impingement, which can cause pain and may lead to OA,49,50 can significantly affect normal hip biomechanics during gait as the result of jamming of the femoral head into the acetabulum.51,52 Patients with FAI have demonstrated decreased frontal and sagittal hip ROM during gait.52
Impediments to normal gait, function, and range of motion often lead to total hip replacement (THR) or hip resurfacing in an attempt to restore daily functional activities.53 Preoperatively, THR patients exhibit slower ambulatory speeds, decreased cadence, and shortened stride length as a result of reduced hip flexion during contact, and reversal of motion during extension at the end of the stance phase.48,54-56 This reversal is caused by flexion contracture, which could reflect enhanced lumbar lordosis and lack of overall hip extension, as well as serving as a method to avoid pain by decreasing hip joint force.48 Hip extension failure during late stance also contributes to decreased step length during gait. Changes in joint geometry can alter muscle strength and the ability of muscles to generate moments.1 The head-neck angle, neck length, and joint center position play a significant role in abductor muscle function, with a varus hip (decreased head-neck angle) providing greater abductor muscle strength, decreasing contact forces, and increasing femoral head and acetabular congruency.1,57 Increased femoral neck length and a more distal greater trochanter position have been shown to clinically increase abductor/adductor strength.58
Adding to these adaptations, the disease process itself alters properties of the hip joint with thickening of the capsule and hip joint effusion, leading to increased intracapsular pressure59 with flexion, stretching of the joint capsule, and significant joint pain.59–61 Further degeneration of the capsule increases stress on surrounding articular cartilage and continues OA-related pain. Studies also suggest that progressive loss of hip extensor strength leads to pathophysiologic complications such as muscle atrophy, buildup of connective tissue and adipocytes in muscles, and potential changes in hip torque-angle relationships.61,62 Consequently, OA consistently remains a source of limitation in hip motion during gait, and studies have well documented the debilitating effects of OA through the impact of pain on physical function.7,61,63
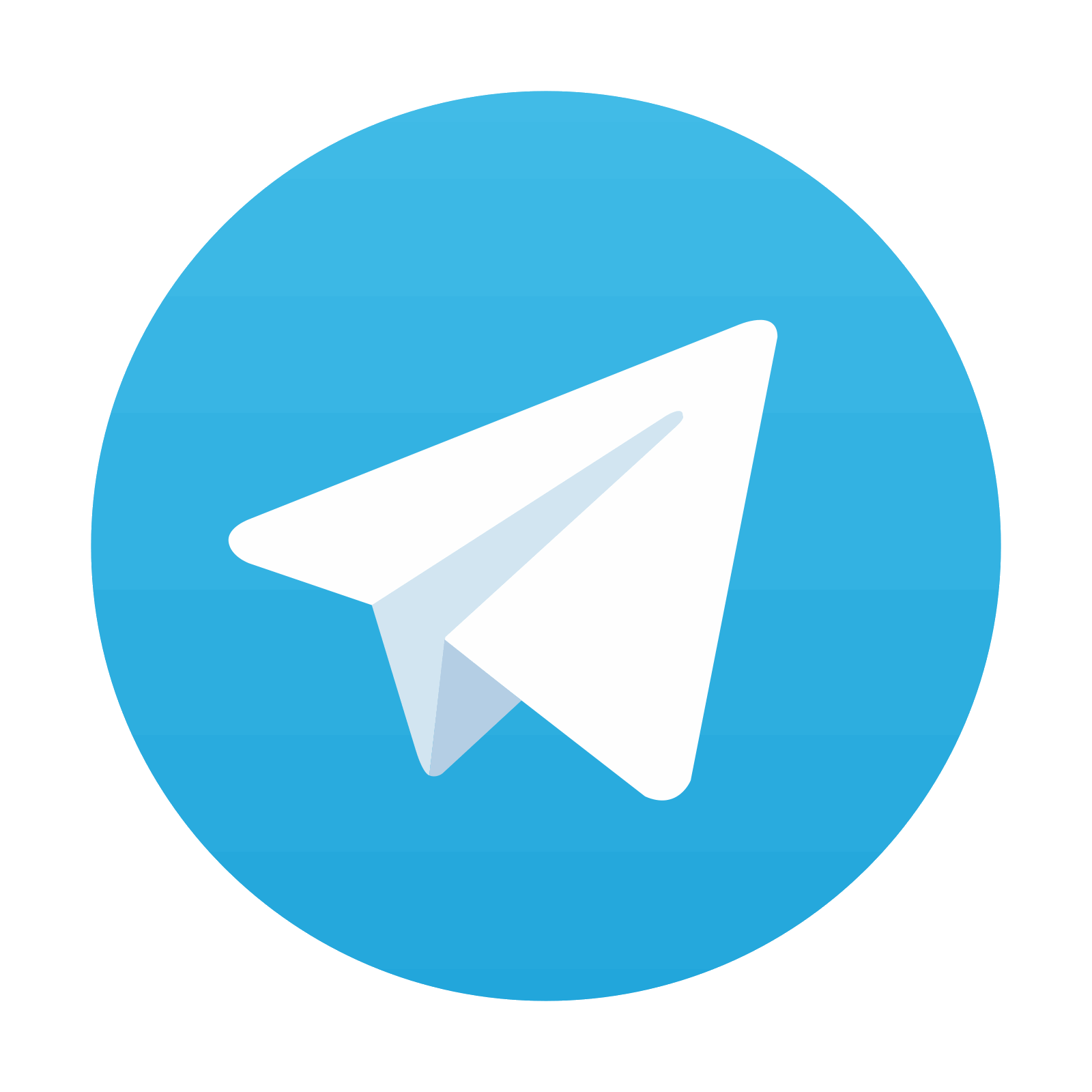
Stay updated, free articles. Join our Telegram channel
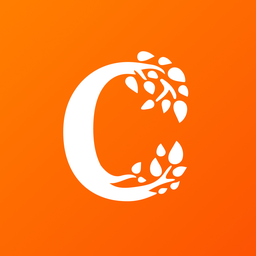
Full access? Get Clinical Tree
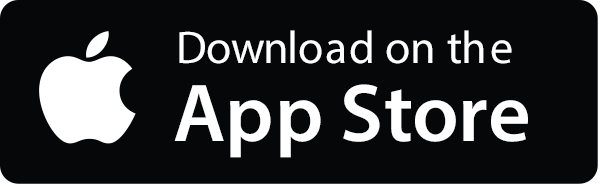
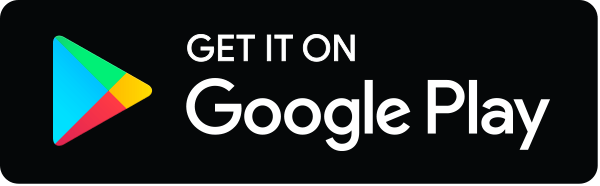