Collagen type
MW
Quantity
Location in the tendon
Type I
95,000
97–98%
Collagen fibers, endotenon, epitenon, paratenon, MTJ
Type II
95,000
0.2–0.8%
Cartilaginous area of OTJ
Type III
95,000
1–1.5%
Endotenon, paratenon, vessel walls, MTJ
Type IV
180,000
<0.2%
Basal membrane of vessels, MTJ
Type V
300,000
<0.2%
vessel walls, MTJ
Table 21.2
Principal component of tendons ECM
Collagen: 86% (type I: 98%) |
Proteoglycan: 1–5% |
Elastin: 2% |
Decorin: <1% |
Aggrecan: <1% |
Other proteins: <1% |
Proteoglycans are the structures principally responsible for the visco-elasticity of tendons. They are macro-molecules composed by a protein core which is connected, through covalent bonds, to long chains of disaccharides and GAGs. In addiction, they form complexes, through non-covalent bonds, with a single molecule of hyaluronic acid.
Adhesive glycoproteins, such as fibronectin and thrombospondin, participate during the repair and regeneration processes of tendons [9–11]. “Tenascin-C,” another important component of the tendon extracellular matrix network, is abundant in the tendon’s body and at the osteotendinous and myotendinous junctions [12, 13]. Tenascin-C contains a number of repeating fibronectin type III domains and, following a stress-induced unfolding of these domains, it also functions as an elastic protein [13, 14]. The expression of tenascin-C is regulated by mechanical strain and it is upregulated in tendinopathy [12, 15, 16]. Tenascin-C may play a role in collagen fiber alignment and orientation.
Among the proteoglycan complexes, it is possible to find collagen, elastin, and fibronectin organized in a system which gives mechanical resistance to the tissue. Collagen molecules are made of polypeptid chains forming a tropocollagen helix, and five of these create a micro-fibril, which unites into fibrils. Fibrils are, then, grouped in fibers (primary bundles) which are organized in fascicles (secondary bundles) [17, 18] (Fig. 21.1).


Fig. 21.1
Structural organization of tendons (Maffulli N, Renström P, Leadbetter WB, editors. Tendon Injuries. Basic Science and Clinical Medicine. London: Springer 2005, pp. 70–86)
A collagen fiber is the smallest tendon unit that can be tested mechanically and it is visible under light microscopy.
At the myotendinous junction, tendinous collagen fibrils are inserted into deep recesses formed by myocyte processes, allowing the tension generated by intracellular contractile proteins of muscle fibers to be transmitted to the collagen fibrils [19, 20]. This complex architecture reduces the tensile stress exerted on a tendon during contraction [19]. However, this junction still remains the weakest point of the muscle-tendon unit [19–21].
The osteotendinous junction is composed of four zones: dense, fibrocartilage, mineralized fibrocartilage, and bone [22]. The specialized structure of the osteotendinous junction prevents collagen or fiber bending, fraying, shearing, and failure [23, 24].
To simplify the sliding and to produce a channel for blood vessels, there is a very thin structure of connective tissue called “endotenon,” interposed between fibers [25]. The endotenon continues in a sheet of connective tissue called “epitenon,” which externally surrounds the tendon, containing the vascular, lymphatic, and nerve supplies of the tendon. Some tendons present another structure of covering called “paratenon,” which covers the epitenon: it is a loose areolar of connective tissue consisting of type I and type III collagen fibrils, some elastic fibrils, and an inner lining of synovial cells [5].
Tendon sliding is supported by a substance interposed between tendon and paratenon called “lubricin.”
Synovial tendon sheaths are found in areas subjected to increased mechanical stress, such as hand and feet’s tendons, where an efficient lubrication is required. Synovial sheaths consist of an outer fibrotic sheath and an inner synovial sheath, which consists of thin visceral and parietal sheets [26]. The inner synovial sheath invests the tendon body and functions as an ultrafiltration membrane to produce synovial fluid [27]. The fibrous sheath forms condensations called “pulleys,” which function as fulcrums to aid tendon function [28].
21.2.2 Vascularization
The vascularization of tendons is an important physiological and pathological area of concern. It can be studied through different methods: intravascular injection of colorants, using a marker for the lamin, instrumental methods (such as Doppler ultrasonography), and histological analysis.
Tendons have a lower blood supply compared to muscles, which have major metabolic activity. For this reason, tendons are white and muscles are red. The presence of vessels in tendons aids to preserve TCs’ function and regenerative capacity.
There are three main systems to receive blood: two intrinsic systems (at the osteotendinous and miotendinous junction) and one extrinsic system (between the paratenon and the synovial sheath) [29, 30]. The ratio between systems varies from tendon to tendon. Vessels arise from the muscles and then extend to the miotendinous junction at the proximal third of a tendon, while those which arise at the osteotendinous junction are limited at the insertion of tendon connected with the periostial vessels [29].
For example, the Achilles tendon receives its blood supply from the vessels of the paratenon (arising from the posterior tibial artery), in its proximal area by vessels originated from the body of the muscle, and in the distal area by vessels arising from the periosteum of the calcaneus. The medial region has a lower blood supply, and that is possibly why it is more vulnerable to rupture.
In tendons enveloped by sheaths to reduce friction, branches from major vessels pass through the vincula (mesotenon) to reach the visceral sheet of the synovial sheath, where they form a plexus [26] which supplies the superficial part of the tendon, while some vessels from the vincula penetrate the epitenon. These penetrating vessels form a connection between the peritendinous and intratendinous vascular networks.
Without a synovial sheath, the paratenon provides the vascular extrinsic component, and the vessels entering in the paratenon course transversely form a complex vascular network. Then, the branches penetrate the epitenon and course in the endotenon septa where they form abundant anastomoses with the intratendinous network [31, 32].
Vascularization is compromised in the junctional zone and in the torsion, friction, and compression sites. Vascularization tends to decrease with the increment of mechanical load and age [33]. In these areas, angiogenesis can be blocked by the expression of inhibitor molecules, such as endostatin, and by a reduction of expression of stimulatory peptides, such as VEGF.
21.2.3 Innervation
Tendons innervation arises from cutaneous, muscular, and peritendinous nerve trunks; fibers form rich plexuses in the paratenon, then branches penetrate the epitenon. Most nerve fibers do not actually enter the main tendons body, but terminate as nerve endings on its surface. At the myotendinous junction, nerve fibers cross and enter the endotenon septa.
The myelinated fibers have principally a mechanical-receptor function, specialized in detecting pressure and tension changes. This is possible thanks to tendon’s Golgi organelles, which are very abundant at the muscle’s insertion site of tendons [34, 35]. They are essentially a thin delicate capsule of connective tissue that encloses a group of branches of large myelinated nerve fibers and terminate with a spray of fiber endings between tendon’s bundles of collagen fibers [36, 37].
Regarding the unmyelinated nerve ending, they act as nociceptors, detecting and transmitting pain. Both sympathetic and parasympathetic fibers are present in tendon [38].
21.2.4 Physiologic Response of the Tendons
The mechanical behavior of collagen depends on the number and types of intramolecular and intermolecular bonds [39]. A stress-strain curve helps to demonstrate the behavior of tendon. The collagen fibers and fibrils display a crimped configuration [40]. The initial concave portion of the curve (toe region), where the tendon is strained up to 2%, represents flattening of the crimp pattern [41–43]. Beyond this point, tendons deform in a linear fashion as a result of intramolecular sliding of collagen triple helices, and the fibers become more parallel [44, 45]. If the strain remains <4%, the tendon behaves in an elastic fashion and returns to its original length when unloaded [46]. Microscopic failure occurs when strain exceeds 4%. Beyond 8% to 10% strain, macroscopic failure occurs from intrafibril damage by molecular slippage [42, 47, 48].
Studies with the X-ray have established that collagen fibril elongation initially occurs as a result of molecular elongation, but as stress increases, the gap between molecules increases, eventually leading to slippage of lateral adjoining molecules [49]. After this, complete failure occurs rapidly, and the fibers recoil into a tangled bud at the ruptured end [2].
The tensile strength is related to thickness and collagen content, and an area of 1 cm2 is capable of bearing 500–1000 kg3 in the tendon [25, 50, 51]. During strenuous activities such as jumping, very high loads are placed on tendons [52]. Forces of 9 kN, corresponding to 12.5 times the body weight, have been recorded in the human Achilles tendon during running [53, 54]. The rate of loading may also play an important role in tendon rupture [36, 54]. Tendons are at the highest risk for rupture if the highest forces are seen during eccentric muscle contraction and the tension is applied quickly and obliquely [39, 55, 56].
Exercise increases the turnover of mature collagen and density, diameter, and rigidity of the fibers, in addition to increasing the galactosamine content of GAGs. Tension and compression forces have a different impact on the expression of proteoglycanes in the tendons: tension induces the synthesis of decorin, the most predominant proteoglycan which provides cross-links between collagen fibers, while compression forces induce the production of aggrecan.
Adaptation to the exercise is characterized by the release of pro-inflammatory molecules, such as IL-1B, and growth factors to regulate cell activity. Exercise, in the long term, reinforces the tendons, increasing the production of new collagen, modifying the proteoglycans content, and improves the tenso-elastic properties.
Adaptation of tendons to training is slower compared to adaptation to de-training. Furthermore, sudden de-training produces typical morfo-structural alterations, which can be dangerous for tendons’ health. For this reason, rehabilitation after an injury must be gradual.
21.3 Part III Tendon Injury
21.3.1 Epidemiology
Tendons can undergo degenerative and traumatic processes. In the United Kingdom, soft tissues disorders have a prevalence of 18 cases for 1000 habitants and represent the causes of 40% of new rheumatologic consultations. However, these data underestimate the problem, because many patients are old, and they do not consult a doctor.
The most vulnerable tendons are those of rotator cuff, the long head of the biceps, the wrist extensors and flexors, the adductors, posterior tibial tendon, the patellar tendon, and the Achilles tendon. At least 50% of tendon problems are secondary to overload.
In particular, the Achilles tendon injuries are common in football, tennis, badminton, and jumping and have a prevalence in running athletes of 11%, but 1/3 of patients with this pathology do not practice intensive physical activity.
Pathology of the extensor tendons of the forearm occurs in 1–2% of the adult population and is very frequent in tennis, baseball, and golf players.
Pathology of the patellar tendon is common in jumping sports such as basketball, tennis, football, hockey, and volleyball.
Rotator cuff disease increases with age. Studies on cadavers have established that the prevalence of this disorder ranges from 30 to 50% in subjects over 70 years of age. Furthermore, it is frequent in throwing sports such as baseball, tennis, volleyball, and javelin.
21.3.2 Tendon Rupture
Tendon rupture is defined as the partial or full thickness tearing of tendon fibers [57]. This condition can be either acute, in the case of an individual experiencing a rupture from a single high-load impact (for example, an Achilles tendon rupture associated with sudden or violent dorsiflexion of ankle or lunge), or chronic, when the tendon is weakened due to tendinopathy or aging, and the tendon ruptures at lower loads [58].
The location of rupture varies with tendon type. For example, the supraspinatus tendon commonly tears at the insertion because of the complex loading environment. However, the Achilles tendon commonly tears at the mid-substance [57].
An acceleration-deceleration mechanism has been reported in up to 90% of sports-related Achilles tendon ruptures [59]. Malfunction of the normal protective inhibitory pathway of the musculotendinous unit may result in injury [60].
The etiology of tendon rupture remains unclear [8]. Degenerative tendinopathy is the most common histological finding in spontaneous tendon ruptures. Tendon degeneration may lead to worsening of mechanical properties, predisposing an individual to increased risk of rupture [57]. Indeed, histological evaluation of ruptured Achilles tendons has demonstrated greater degeneration than that found in tendons that were chronically painful as a result of an overuse injury [61].
Unfortunately, after rupture, tendons generally form scar tissue during healing, and most will never regain the same collagen structure, composition, and organization of healthy tissue. This can lead to a decrease in the mechanical properties of the tissue and the increased potential for re-rupture [62].
21.3.3 Tendinopathies
21.3.3.1 Terminology
In the past, “tendinitis” was used to describe a condition characterized by chronic pain in the tendon region, emphasizing the inflammatory process which was thought to be at the basis of the disorder.
The terms “tendinitis” and “tendinosis” are suggested to be used only after histopathological examination [63]: the first assumed to be accompanied by inflammation and pain, whereas the second can be caused by tendinous degeneration without the presence of evident inflammation.
Histological studies have described the presence of failed healing lesions without or with only a minimal presence of an inflammatory component. The normal architecture of the collagen is replaced by a mucinous amorphous material and other modifications that will be described in this chapter.
Therefore, the term “tendinopathy” includes all the situations in which there are clinical conditions characterized by pain, swelling, and functional limitations of tendons and nearby structures.
The term “tendinopathy” is recommended to be used as a generic descriptor of the clinical conditions in and around tendons arising from overuse. It includes both microscopic and macroscopic abnormalities found in tendon, and it is not uncommon for several pathological conditions to coexist in tendinous structures [64, 65].
It is believed that these conditions are rarely spontaneous and are not caused by single factors. Rather, they are the end result of a variety of pathological processes which can ultimately lead to the main clinical problem: loss of tissue integrity with full or partial rupture of the tendon.
Furthermore, a tendinopathy may be classified on the basis of the specific structures involved, as reported in the Table 21.3.
Table 21.3
Terminology of tendinopathy (Maffulli N, Khan KM, Puddu G. Overuse tendon conditions: time to change a confusing terminology. Arthroscopy 1998;14:840–3, with permission)
Tendinopathy of tendons body |
Tendinopathy of adjacent tissues |
Pantendinopathy |
Insertional tendinopathy |
Tendons ruptures |
21.3.3.2 Etiology
At least 50% of all injuries sustained during sport activity are related to overload. This causes repetitive microtrauma on the tendon which can occur from stresses within the physiological limits [66] of the tendon itself. The subclinical damages can accumulate before the tendon becomes symptomatic with pain. The specific mechanisms are not clear, but, on the bases of experimental and clinical studies, some light has been shed on the etiopathogenesis of tendinopathy.
Tendon injuries can be acute or chronic and are caused by intrinsic or extrinsic factors, either alone or in combination. In acute trauma, extrinsic factors predominate, while in chronic cases intrinsic factors also play a role. These factors are associated with the onset of overload pathology of tendons, though there is no specific relationship between cause and effect. The intrinsic factors are represented by limited flexibility, muscle weakness, and joint instability. The extrinsic factors are incorrect sport technique, inappropriate equipment, and use of drugs (such as fluoroquinolones; Table 21.4). Interaction between intrinsic and extrinsic factors is common in chronic tendon disorders [8].
Table 21.4
Intrinsic and extrinsic factors
Intrinsic factors |
• Age |
• Body habitus |
• Metabolic diseases |
• Chronic Kidney failure |
• Nutrition |
• Genetics |
Extrinsic factors |
• Drugs (fluoroquinolones) |
• Excess loading |
• Fatigue loading |
• Improper loading |
• Disuse |
• Compression |
• Exogenous damage (tobacco, direct injury) |
Intrinsic Factors
Intrinsic factors directly affect the health and composition of tendon from the local environment in which it forms, that is the person’s body. These intrinsic factors can affect tendon’s health, strength, and composition in many ways:
Age: cells frequently shift from aerobic to more anaerobic energy production, increasing the matrix metalloproteinase (MMP) production, leading to an imbalance between tendon destruction and composition, with an increase in matrix degeneration [67]. In addition, cells become less plastic and vascular supply to the tendon structure is decreased [68].
Body Habitus: Heavier patients, by definition, place a greater load on weight-bearing tendons while walking, subjecting the structure to a higher strain. Regardless of the overall strength of the tendon, repetitive strain approaching the ultimate load-to-failure point of the tendon causes mechanical deformation and a higher risk of rupture. Injuries can also result from a sudden increase in repetitive workload without sufficient time to condition tendons and allow for cell turnover and injury repair. In this way, tendon may undergo further injury with progressively worsening tendinopathy.
Morphological differences between patients can also predispose certain individuals to tendon injury. Many of these conditions are not considered pathological disorders, but merely anatomic variations [69]. For example, acromial types include flat, curved, and hooked, and excessive stressis can be placed on the underlying rotator cuff tendons during activities of daily living. Evidence shows the hook type predisposes individuals to a higher rate of rotator cuff tendinopathy and tears at earlier ages [70].
Nutrition: While ischemia is tolerated for a relatively long time to allow for long periods of loading and tension, prolonged hypoxic stress can cause decreased cell function and eventual death. In addition, prolonged hypoxia can lead to reperfusion injury, which increase the free radicals, leading to increased oxidative stress that creates tissue damage.
Metabolic Diseases: Systemic comorbidities may cause injury to tendons and can occur by directly affecting the tendon or causing an alteration in the local growth environment [71]. For examples:
Diabetes is well-documented to be associated to tendinopathy. It can alter the cellular metabolism, increase intracellular water and edema, and decrease the ability of cells to undergo growth and tolerate ischemic and oxidative stress. Furthermore, the advanced glycosylation end-products are increased and, when deposited, form cross-links within collagen fibers, altering their structure, increasing pro-inflammatory pathways, and modifying proteins involved in cellular regeneration.
Dyslipidemia may cause disorganization of collagen fibrils and decrease their density, resulting in decreased overall strength.
Hypercholesterolemia can occur macroscopically as an abnormal fat deposition in tendons, producing “tendon xanthomas.”
Calcific tendinopathy is the deposition of calcium directly in tendons, frequently seen in the rotator cuff tendons. It is unknown whether this is a cause of tendinopathy itself or results from tendon injury and is an aberrant response by the TCs.
Inflammatory arthropathies such as rheumatoid and psoriatic arthritis can cause direct damage and inflammation of tendons and impede healing.
Genetics: Recent evidence suggests that genetics plays a major role in both tendon strength and ability to resist and repair injury. These are multifactorial, and different structures may depend on different genetic loci; variations may be seen in normal population variance or as part of distinct clinical pathologies/disorders [72, 73].
Extrinsic Factors
Extrinsic factors of tendinopathy influence the tendon itself from the outside. The most important are:
Excess Loading: it can cause microscopic damage to the tendon. As strain increases, initially the tendon fibrils are straightened and undergo a little added strain with increasing stress [74]. Once the tendon is stretched, the intrinsic stiffness of the tendon is represented in the linear region. In this region, stiffness can be calculated and differs for each substance. Depending on the tendon’s composition, organization, and structure, the modulus can vary greatly.
Fatigue Loading: Injury can also result from repetitive loading within the physiological range. Repeated microtrauma can cause stress and altered mechanical properties. Mechanical loading of the tendon within physiological limits stimulates fibroblast proliferation and collagen synthesis and realignment, promoting proper organization. If the tendon is subjected to repeated trauma without sufficient time for repair or without adequate biological mechanisms to undergo regeneration, the cumulative effects may lead to tendinopathy and eventual rupture.
Improper Loading: It results when an abnormal load is seen in the tendon because of abnormal positioning. Eccentric positioning of the forces acting on a tendon can result in frictional forces between the fibrils and cause microtrauma and direct damage. This can result in abnormal healing and localized fibril damage and disorganization. Degeneration and disorganization leads directly to reduced tensile strength and may result in abnormal healing and an increased predisposition for rupture [75].
Disuse: Without physiological levels of stress, tendons undergo degeneration and show a decrease in mechanical properties [76]. Microscopic changes include a decrease in cellular number and tensile modulus, more disorganized collagen, and changes in cellular morphology and reparative ability [77]. In canine models, the disuse has shown to cause a decrease in collagen types I–III, aggrecan, decorin, and fibronectin [78].
Compression: While tendons typically undergo tensile stresses, compressive forces are also seen in some areas [79]. The bone tendon junction has a transition zone where tendon interdigitates with fibrocartilage, which is mineralized and connects to bone. In this area, the tendon experiences compressive load, and most clinical tendinopathy occurs. Altered loading can increase compressive forces, necessitating the upregulation of fibrocartilage formation. This can cause decreased tissue properties and predispose the structure to tendinopathy [80].
Exogenous Damage: it can occur from sources outside of the body, either systemically or from local effects. For example:
Fluoroquinolones: drugs that may impede cellular turnover and ability of heal and have a cumulative effect,
Tobacco: hampers the ability to heal and remodel in the setting of microtrauma, even when tendons are subjected to a physiological load.
Direct mechanical injury: represents a large proportion of clinical tendon injury. Tendon lacerations are frequently divided into partial and complete tendon lacerations, for which there is considerable controversy regarding fixation guidelines. Blunt trauma without penetration or laceration, despite the viscoelastic properties and relative tensile strength of tendons, can cause rupture [81].
21.3.3.3 Histological Characterization
When there is a repeated overload, the collagen fibers begin to slither between them, breaking their covalent bonds determining a denaturation of the tendon tissue; furthermore, the ECM and vessels are altered. The histological aspects are represented by:
Non-inflammatory intratendinous degeneration of collagen fibers, altered orientation with reduced thickness of the fibers, scattered vascular ingrowth, increased production of collagen of type III and interfibrillar glycosaminoglycans [26, 82, 83];
Variability of cell density, with areas in which there are lot of rounded tenocytes, which have a chondroid appearance, and areas poor of tenocytes which have a small and pyknotic nuclei;
Increasing of ECM, disordered and haphazard healing with an absence of inflammatory cells, and poor healing response without inflammatory signs. Frank inflammatory lesions and granulation tissue are infrequent and are mostly associated with tendon ruptures [84].
Various types of degeneration may be seen in tendons, but mucoid or lipoid degeneration is usually found in the Achilles tendon [26, 85]. Light microscopy of a tendon with mucoid degeneration reveals large mucoid patches and vacuoles between fibers. In lipoid degeneration, abnormal intratendinous accumulation of lipid occurs, with disruption of collagen fiber structure [26, 86, 87]. In patellar tendinopathy, mucoid degeneration is commonly seen, although hyaline degeneration rarely occurs [88, 89]. In rotator cuff tendinopathy, mucoid degeneration occurs, but fibrocartilaginous metaplasia, often accompanied by calcium deposition, is also common [90]. Amyloid deposition in supraspinatus tendons with degenerative tears has also been reported [91].
Tendinopathy can be viewed as a failure of the cell matrix to adapt to a variety of stresses as a result of an imbalance between matrix degeneration and synthesis [67, 92]. Macroscopically, the affected portions of the tendon have lost their normal glistening-white appearance and have become gray-brown and amorphous. Tendon thickening, which can be diffuse, fusiform, or nodular, occurs [93]. Tendinopathic changes are often clinically silent, and the only manifestation may be a rupture; however, they may also coexist with symptomatic paratendinopathy [94–96]. Mucoid degeneration, fibrosis, and vascular proliferation with a slight inflammatory infiltrate have been reported in paratendinopathy [8, 97, 98]. Edema and hyperemia of the paratenon are seen clinically. A fibrinous exudate accumulates within the tendon sheath, and crepitus may be felt on clinical examination [93].
In samples from 397 ruptured Achilles tendons, Kannus and Jozsa [6] found no evidence of inflammation under light and electron microscopy. Arner et al. [100] also found no neutrophilic infiltration in Achilles tendons on the first day after rupture, and they concluded that any inflammation seen at a later stage occurred subsequent to the rupture. In a recent study, immunohistochemical staining of neutrophils confirmed acute inflammation in all of 60 ruptured Achilles tendons [100]. Collagen degeneration and tenocyte necrosis may trigger an acute inflammatory response, which further weakens the tendon, predisposing it to rupture.
In summary, tendinopathy shows features of disordered healing, and inflammation is not typically seen. Although degenerative changes do not always lead to symptoms, preexisting degeneration has been implicated as a risk factor for acute tendon rupture [6, 61, 99]. The role played by inflammation in tendon rupture is less clear.
The underlying pathophysiological process that results in tendinopathy also remains undetermined. It is possible that there may be several pathways that can result in tendon degeneration. The following mechanisms have all been proposed as initiators of tendinopathy: hypoxia, ischemic damage, oxidative stress, hyperthermia, impaired apoptosis, inflammatory mediators, fluoroquinolones, and matrix metalloproteinase imbalance [8, 13, 26, 41, 44, 101].
21.3.3.4 Molecular Characterization
Tenocytes can modulate their activity in response to the modification of mechanical loads: in fact, as established in vitro, traction forces can increase the collagen synthesis. This process involves two types of cell junctions characterized by the presence of connexin 32 and connexin 43: while the first increases the synthesis of collagen, the second reduces it. Junctions that express both of connexins link tenocytes in a single longitudinal row; lateral junctions between cells only express connexins 43. Therefore, connexin 32 is present on the principal stress lines of traction in tendons, while connexin 43 is present in all directions. It is possible that these two networks of communication have different functions: tenocytes may have a basal level of synthesis, amplificated by connexin 32, and at this point, the signal of connexin 43 may become active, attenuating the response to the mechanical stress and maintaining control.
Beyond the effects on the collagen synthesis, stretching in tenocytes, in vitro, increases the production of pro-inflammatory cytokines and the expression of mediators such as COX-2, PGE2, MMP-1, and IL-1β. It is known that lower repetitive traction stress levels reduce the production of pro-inflammatory molecules. That is why small stretching entities seem to have an anti-inflammatory effect.
The meaning of the production of IL-1β is the induction of the expression of pro-inflammatory molecules such as COX-2 MMP1, MMP3, MMP13, ADAMTS-4, IL-6, through which the destruction and modification of the ECM increases. In this case, there is inability of adaptation of the ECM to the mechanical stress. Corticosteroids, which reduce COX-2 and MMP expression, can reduce, in vitro, proteoglycans and collagen synthesis: if this effect happens in vivo, it could explain why tendons’ health could be compromised by the use of these drugs.
When a tendon is subject to maximal tension, ischemia may occur, and some tendons have more sensibility (e.g., Achilles tendon and posterior tibial). During the relaxing phase, there is a reperfusion which produces free radicals that can cause tendinopathy. Normally, tenocytes express an antioxidant enzyme, called peroxiredoxin 5, which protects cells against damage from different reactive oxygen species, and during an ischemic period its expression increases. Hypoxia also increases the expression of VEGF and MMP which impairs tendon structure.
During movements, a 5–10% of energy produced by tendons is converted in heat. It is possible that the inability to control exercise-induced hyperthemia can determine tenocytes’ death. It is uncommon that a single episode can lead to this effect, but repeated hyperthermic injuries and protracted hyperthermia can determine it.
In the genesis of tendinopathy, the excessive apoptosis of tenocytes can be implicated. Oxidative stresses can activate the production of stress-inducted kinases such as c-Jun N-terminal kinases (JNK) and Caspase-3, which induce apoptosis; the exact phenomena are still unclear [102]. For example, tendinopathic quadriceps femoris tendons exhibit a rate of spontaneous apoptosis that is 1.6 times greater than that of normal tendons [103].
Ciprofloxacin also induces IL-1β-mediated MMP-3 release, while the use of fluoroquinolone is associated with tendon rupture and tendinopathy [104, 105]. The mechanism that can lead to tendinopathy mediated by the fluoroquinolones could be the inhibition of tenocyte metabolism, reducing cell proliferation, collagen, and matrix synthesis [106, 107].
MMPs, a family of proteolytic enzymes, have both the ability to degrade the components of the extracellular matrix network and facilitate tissue remodeling [108, 109]. Many studies have reported a downregulation of MMP-3 mRNA in Achilles tendinopathy and an upregulation of MMP-2 (gelatinase A) and VEGF in Achilles tendinopathy compared with control samples [110, 111]. In supraspinatus tendon rupture, an increase of MMP-1 (collagenase-1) activity and a TIMP-1 expression have been reported [67, 112].
An imbalance in MMP activity has been demonstrated in tendinopathic and ruptured tendons, and differences in MMPs expression have been reported [108, 112]. A differential temporal sequence of MMPs expression may occur, and MMPs expression may differ between tendinopathic and ruptured tendons.
IL-33 is a member of the IL-1 cytokine family and is released following cellular damage [113] and biomechanical overload [114], functioning via its cognate receptor ST2. Millar et al. [115] found that miR-29a acts as a critical regulator of tenocyte biology by integrating IL-33 effector function and collagen matrix changes. Tendon injuries or repetitive micro-tears causing stress of tendon cells result in the release of IL-33, which downregulate the expression of miR-29a through NFkB phosphorylation and produce ST2, triggering inflammation and switching collagen production towards biomechanically inferior collagen III synthesis which reduces the tendon tensile strength, propelling the tendon towards a pathological state such as that seen in early tendinopathy biopsies. However, elevated ST2 may act, in a feedback loop fashion, as a protective mechanism by removing excess IL-33 from the system. For this reason, they consider IL-33 as an influential alarmin which may be important in the balance between reparation and degeneration.
Another important molecule is nitric oxide (NO), which has a short half-live and is also bactericide, anti-angiogenic (at high concentrations), and stimulates vasodilation and local perfusion. The NO synthase (NOS) starts from arginine, which is induced by mechanical activity and moderate physical activity. Experimental and clinical data have shown an increase in ECM synthesis and an improvement of the mechanical propriety of the injured tendons. For this reason, the NO may play an important role in tendon healing, but there are no evidences, in clinical practice, that suggest a certain effect in patients with this pathology.
21.3.3.5 Clinical Characterization
Pain is associated with inflammation, but in the tendinopathy there is little patient evidence of inflammation. Pain is probably originated from a combination of mechanical and biochemical factors [82].
Tendon degeneration associated to a mechanical breakdown of collagen could theoretically explain the pain, but clinical and surgical observations have challenged this point of view [82]. Microdialysis sampling has revealed a twofold increase in lactate levels in tendons with tendinopathy compared with controls [116].
Patients with chronic Achilles tendinopathy and patellar tendinopathy showed high concentrations of the glutamate neurotransmitter, with no significant elevation of the pro-inflammatory prostaglandin PGE2 [117]. However, levels of PGE2 were consistently higher in tendinopathic tendons than that in control.
Substance P is a neurotransmitter and neuromodulator, and it is found in small unmyelinated sensory nerve fibers [118]. A network of sensitive innervation is present in tendons, and substance P has been found both in tendinopathic Achilles tendons and in medial and lateral epicondylopathies [119, 120]. Increased levels of substance P are correlated with pain in rotator cuff disease [120].
21.4 Part IV: Tendon Healing
Tendon ruptures occur from acute trauma or a chronic degenerative state that weakens the tendon. Once ruptured, the tendon undergoes healing to repair the injured tendon and regain function [57].
The lack of detailed molecular and histopathological studies on tendons has hampered our understanding of the mechanisms underlying tendon healing [123]. Studies of tendon healing predominantly have been performed on transected animal tendons or ruptured human tendons, and their relevance to healing of tendinopathic human tendons remains unclear [58].
21.4.1 Phases
In general, the healing process of an injured or compromised tendon passes through three overlapping phases with distinctive cellular and molecular cascades: (1) inflammation, (2) proliferation, and (3) remodeling [123] (Table 21.5).

Table 21.5
The three phases of tendon healing (inflammatory, proliferation, and remodeling) and their duration over the weeks

21.4.1.1 Inflammation
The inflammatory phase of healing begins immediately after injury and lasts for up to 7 days. Initially, hemostasis occurs, with erythrocytes and platelets entering the injury site. Platelets aggregate and form a fibrin clot to stabilize the injury, while releasing pro-inflammatory cytokines to recruit immune cells, mainly neutrophils [57]. In the first 24 h, monocytes and macrophages predominate, and phagocytosis of necrotic tissue occurs. Then, vasoactive and chemotactic factors are released to initiate angiogenesis, TCs proliferation, and ECM formation [58, 124].
Secreted angiogenic factors initiate the formation of a vascular network, which is responsible for the survival of the newly forming fibrous tissue at the injury site. Despite being profuse and haphazard, the initial vascular response is essential, since it has been shown that a decreased blood supply impairs healing [125].
Next, TCs gradually migrate to the wound and components of the ECM, predominantly collagen type III, are synthesized by recruited fibroblasts [123].
Mechanical stretching is thought to be harmful to healing during this initial inflammatory phase [126].
21.4.1.2 Proliferation
The proliferative phase of healing begins 3–7 days after injury and continues for weeks. During this phase, TCs and macrophages proliferate and initiate tissue synthesis. Production of type I collagen initially decreases, whereas the synthesis of type III collagen peaks during this stage. The ECM becomes filled in with large amounts of collagen arranged in a random manner, as well as non-collagenous proteins, including proteoglycans (PGs) and GAGs, maintaining a high water content in the tissue [57].
21.4.1.3 Remodeling
The remodeling phase of healing begins approximately 6 weeks after injury and lasts around 1–2 years, depending on the age and condition of the patient [58, 123]. This phase can be divided into a consolidation stage and a maturation stage [130].
The consolidation stage begins at about 6 weeks and continues for up to 10 weeks. In this period, the repair tissue changes from cellular to fibrous: there is a decrease in cellularity and matrix production, as the tissue becomes more fibrous through the replacement of collagen type III by collagen type I. Tenocyte metabolism remains high during this period, and TCs and collagen fibers start to organize along the longitudinal axis of the tendon, thereby restoring tendon stiffness and tensile strength [58, 123].
While this increased organization improves the tensile mechanical properties toward normal tendon, the repaired tissue is still fibrous scar and will never completely regain its pre-injury structural, compositional, or functional properties [57].
21.4.2 Chemical Modulation of Tendon Healing Process
Throughout the stages of healing, the tendon healing process is complexly orchestrated by a variety of secreted molecules [134] which play critical roles in the initiation and progression of biological responses. Understanding the roles of each molecule can help to elucidate the underlying mechanism of tendon healing, to glimpse at the mechanisms that cause failed healing responses [57].
21.4.2.1 Molecular Changes
- 1.
During the inflammatory phase, inflammatory cytokines, such as interleukin (IL)-6 and IL-1β, are produced by the invading inflammatory cells. Other molecules involved in this stage are bFGF (basic fibroblast growth factor), IGF-1 (insulin-like growth factor-1), NO (nitric oxide), PDGF (platelet-derived growth factor), PGE2 (prostaglandin E2), TGFβ (transforming growth factor beta), TNF-α (tumor necrosis factor-α), and VEGF (vascular endothelial growth factor) (Table 21.6).
- 2.
During the proliferation phase, many growth factors such as bFGF, BMPs (bone morphogenetic proteins)-12, -13, and -14, also known as GDFs (growth and differentiation factors) -5, -6, and -7, respectively, IGF-1, MMPs, PDGF, substance P, TGFβ, and VEGF are involved (Table 21.6).
- 3.
During the remodeling phase, GDFs -5, -6, and -7 respectively, IGF-1, and TGFβ are involved. (Table 21.6).
Table 21.6
Molecular and histological changes occurring during the three phases of tendon repair
Inflammatory | Proliferation | Remodeling | |
---|---|---|---|
Molecular changes | IL-6, IL-1β bFGF IGF-1 NO PDGF PGE2 TGF-β TNF-α VEGF | bFGF GDF -5, -6, -7 IGF-1 MMP PDGF SP TGF-β VEGF | GDF -5, -6, -7 IGF-1 TGF-β |
Histological changes | Erythrocytes ↑ Platelets ↑ Neutrophils ↑ Monocytes ↑ Macrophages ↑ | Tenocytes ↑ Macrophages ↑ Type III collagen ↑ Proteoglycans ↑ Glycosaminoglycans ↑ | Consolidation stage • Tenocytes ↑ • Type I Collagen ↑ Maturation stage • Tenocytes ↓ • Vascularity ↓ |
IL-1β is a cytokine that promotes inflammation, expression of other inflammatory and catabolic enzymes, degradation of the ECM, and downregulation of type I collagen synthesis. It is dramatically upregulated in the presence of tendon injury. This inflammatory response is necessary for the recruitment of immune cells and tendon fibroblasts to the injury site and for the expression of other important factors, such as TGF-β and PDGF, to promote ECM synthesis [57, 135].
The fibroblast growth factor (FGF), family of cytokines, plays a role in cell migration, angiogenesis, and cell proliferation and is expressed by both fibroblasts and inflammatory cells [57]. FGF-1 and FGF-2, also known as bFGF, are most abundant in adult tendon tissues [136, 137]. Chang et al. [138] found upregulated bFGF mRNA in mature TCs and in fibroblasts and inflammatory cells surrounding the healing site in the tendon sheath. Being elevated early in the healing process [139, 140], bFGF is well-positioned to promote the early events in tendon healing [141].
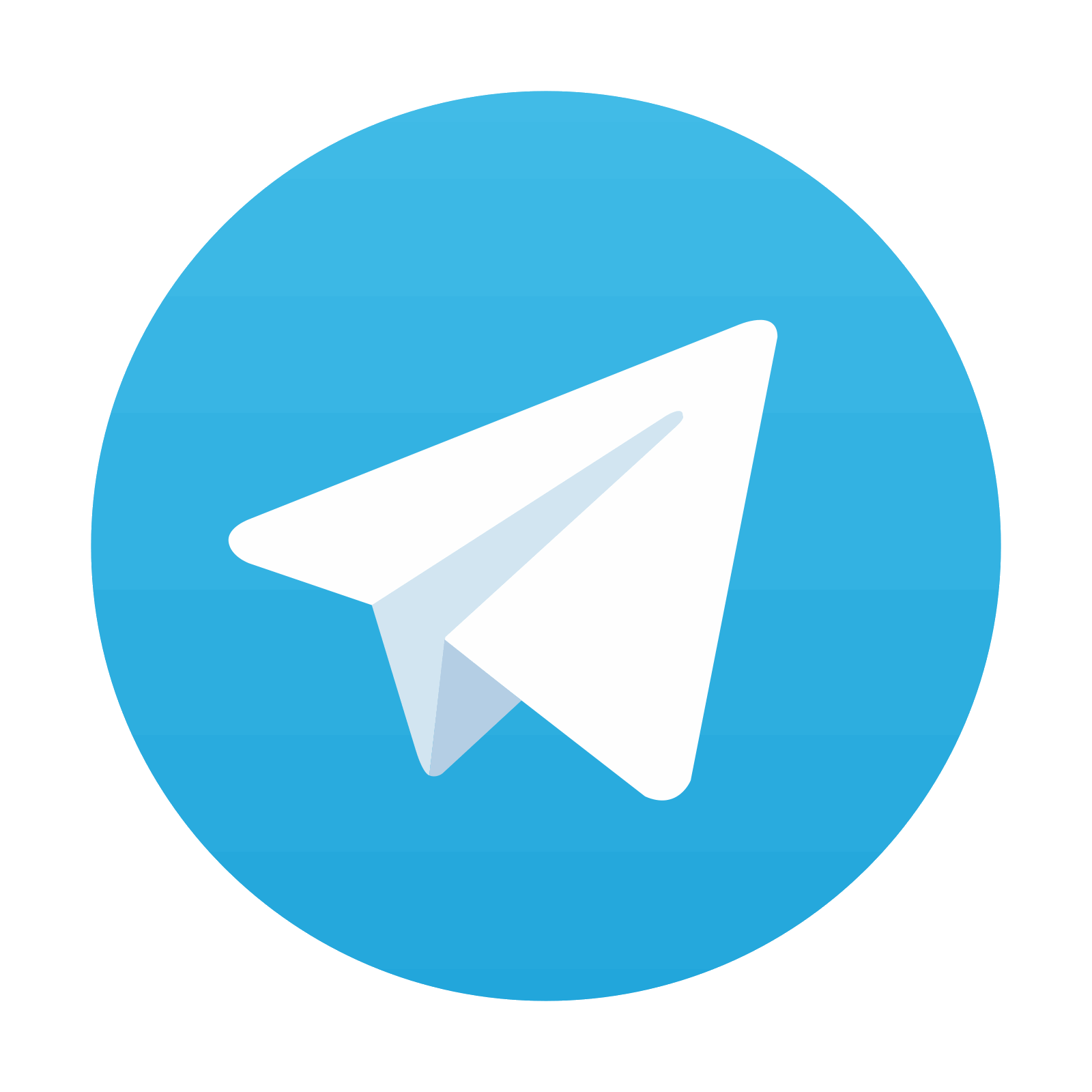
Stay updated, free articles. Join our Telegram channel
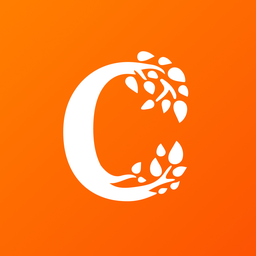
Full access? Get Clinical Tree
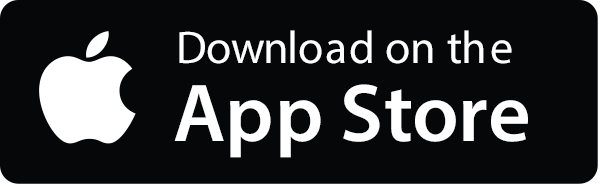
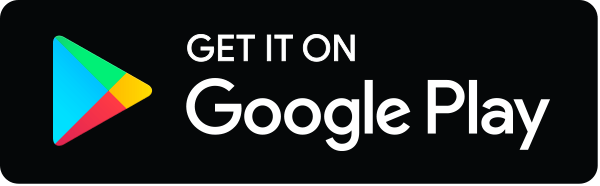