Fig. 10.1
Schematic of a horizontal meniscal tear highlighting the limitations to repair (hypovascularity, hypocellularity, and inflammation) and pro-repair strategies, including enhancing vascularity (angiogenesis, fibrin clot, vascularized graft or conduit), improving cellularity (recruitment or delivery) and generating an instructive environment via biochemical (inflammatory inhibitors and anabolic growth factors) and mechanical cues (dynamic loading)
Vascular Supply and Meniscus Healing
Healing is characterized by cellular invasion into the defect, with subsequent proliferation, ECM remodeling, and synthesis of new matrix proteins to bridge the wound gap. Thus, the availability of a vascular supply that can deliver regenerative cells, nutrients, and growth factors is likely to be crucial to a successful repair response. The region-specific healing that is seen clinically is largely attributed to degrees of vascularity, where limited vascularization precludes the formation of a fibrin clot that stimulates cellular infiltration and matrix remodeling in the inner, avascular zones of the tissue. Since vessels from the perimeniscal capillary plexus fail to penetrate past the peripheral one-third of the meniscus in the adult (red–red zone) [15, 16], this results in an inner avascular zone (white–white zone) with poor healing capacity [17–20]. Junctional tears (red–white zone), as expected, possess intermediate healing capacity. Thus, attempted repair of an avascular tear has a high failure rate and is generally not recommended despite the pathological consequences of partial meniscus removal [21, 22].
Cell and Matrix Density and Meniscus Healing
The presence of a sufficient number of cells at the wound interface is also critical in the healing response. Meniscal cells normally are responsible for maintaining meniscus structure, an active process wherein the ECM is continuously being remodeled due to physiologic loading. These same cells can respond to injury or altered loading by upregulating production of matrix proteins and/or enzymes to affect repair. However, cellularity decreases progressively with maturation until a low cell density is reached in the adult [23, 24] (Fig. 10.2a). Along with a loss in cellularity, meniscus collagen and proteoglycan content increases with age, increasing the overall ECM density and compressive modulus (Fig. 10.2a, b). The lack of viable cell density in older patients renders them more susceptible to meniscal degeneration and re-tear after repair [25] (Fig. 10.2c, d). Furthermore, the increased ECM density likely reduces the capacity of endogenous cells to migrate and remodel the tissue after injury [23, 24]. In addition to changes that occur with maturation, there are also zonal differences of the cells within the meniscus itself. A superior healing response is often seen in the peripheral region, even after eliminating contributions from the microvasculature and synovium, indicating that there may be intrinsic zonal cellular differences that influence repair [26]. Cell morphology and gene expression vary with meniscal region, with fusiform cells located superficially, fibroblast-like cells in the fibrous periphery, and chondrocyte-like cells in the inner fibrocartilage [27–30]. ECM components also differ by region, where the outer portion consists mainly of type I collagen (80 %) to resist tensile stresses and the inner portion has a greater percentage of type II collagen and proteoglycans, which are more resistant to compressive forces [31] (Fig. 10.2a). As a result, there are zonal differences in regenerative mechanisms, sensitivity to cytokines, and response to growth factors [30].
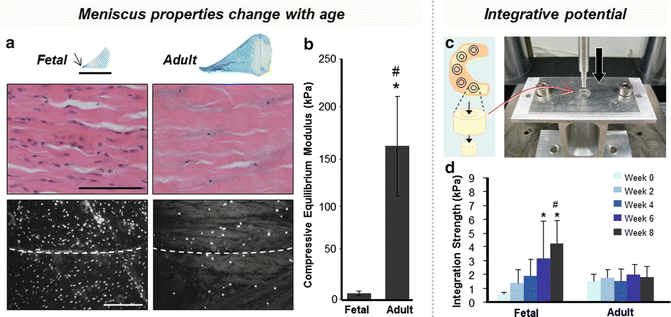
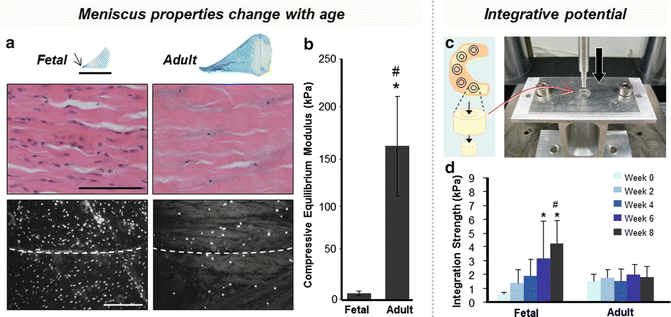
Fig. 10.2
Meniscus properties change with age, contributing to a reduced integrative potential in mature patients. (a) Matrix proteoglycan content and collagen density increase in the adult meniscus (arrow indicates the inner zone’s concentration of proteoglycans) [24], while cellularity decreases compared to fetal meniscus (dashed line indicates wound interface) [127]. (b) The compressive modulus of adult bovine meniscus is greater than that of fetal meniscus [24]. (c) Integration is tested by pushing a cylindrical core out from a surrounding annulus [24]. (d) Integration strength for adult meniscus is lower than fetal meniscus and does not improve with time in culture [24]
Soluble and Mechanical Factors and Meniscus Healing
Meniscal healing is also influenced by soluble and mechanical cues. Inflammatory cytokines that are upregulated post-injury, especially interleukin-1beta (IL-1β) and tumor necrosis factor alpha (TNFα), inhibit integrative repair [32]. Specifically, IL-1β increases the expression of matrix metalloproteinases (MMPs), catabolic enzymes that cleave collagens and other ECM components [33]. On the other hand, cells are stimulated to proliferate and produce matrix when exposed to anabolic growth factors. Mechanical loading, such as static or dynamic compression, can also alter gene expression and modulate inflammation [34, 35]. Thus, introduction of bioactive factors which modulate inflammation and potentiate anabolism and/or loading of the extracellular milieu has the potential to create a matrix-forming environment more conducive to repair.
Emerging Strategies to Promote Meniscus Repair
Numerous techniques have been investigated to address the biologic issues of suboptimal meniscus healing and to improve repair (Fig. 10.1). One approach is to enhance vascularity by increasing access to preexisting vasculature or promoting neovascularization to improve delivery of nutrients, reparative cells, and growth factors to the wound site (Fig. 10.3). Another method is to improve the cellularity of the interface by recruiting endogenous cells or introducing exogenous cells (Fig. 10.4). A related approach is to then encourage the cell population to proliferate and increase matrix synthesis by introducing soluble factors and/or mechanical stimulation that promote anabolic activity and inhibit inflammation (Fig. 10.5). These experimental strategies to enhance integrative repair will be discussed in the following sections.
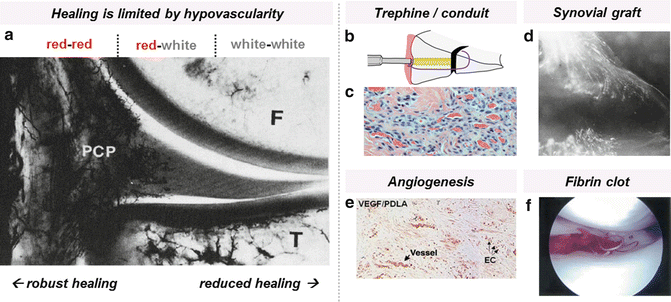
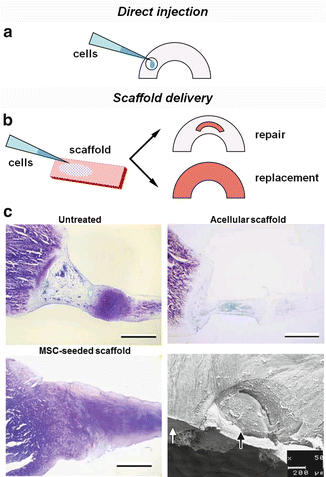
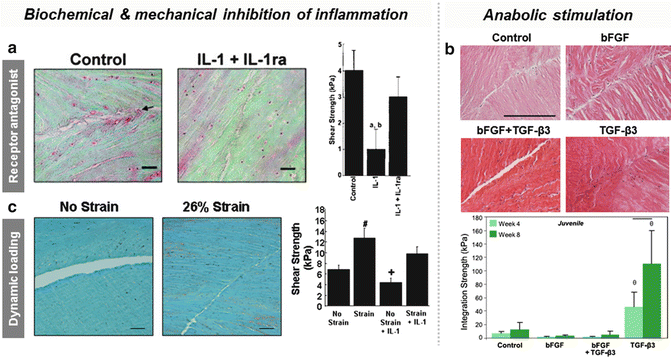
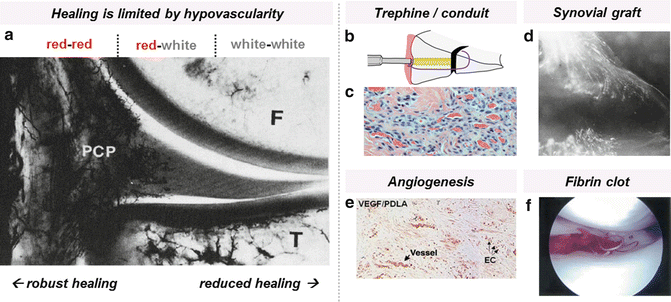
Fig. 10.3
Lack of vasculature in the inner meniscus (white–white zone) results in limited healing after injury. (a) Vascular perfusion shows the perimeniscal capillary plexus (PCP) of the medial meniscus (reprinted by permission of SAGE Publications from reference [16]). Strategies to enhance vascularity include increasing vascular access via a trephine/conduit or synovial graft, promoting angiogenesis, and introducing vascular elements. (b) Trephine/conduit schematic. (c) Tissue and vascular ingrowth using bioabsorbable conduit (reprinted by permission of SAGE Publications from reference [43]). (d) Synovial graft placed above meniscal allograft results in vascularization after 8 weeks as seen by microangiography (reprinted with permission from Springer from reference [47]). (e) Factor VIII immunostaining indicates early vessel formation in meniscus repaired with VEGF-coated sutures (reprinted with permission from Springer Science and Business Media from reference [50]). (f) Fibrin clot placed into meniscal tear with sutures (reprinted with permission from Elsevier from reference [65])
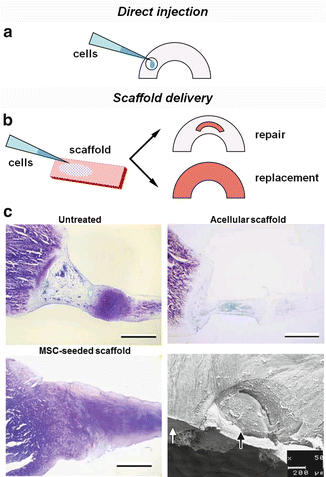
Fig. 10.4
Cellularity can be increased by (a) direct injection of cells into the lesion or (b) delivery via a scaffold. (c) Bone marrow-derived mesenchymal stem cells seeded onto a hyaluronan-collagen composite scaffold improved matrix production and integration in rabbits compared to untreated and acellular groups (reprinted with permission from John Wiley and Sons from reference [95]). SEM image shows the integrated interface of the tissue (white arrow) and pre-cultured scaffold (black arrow)
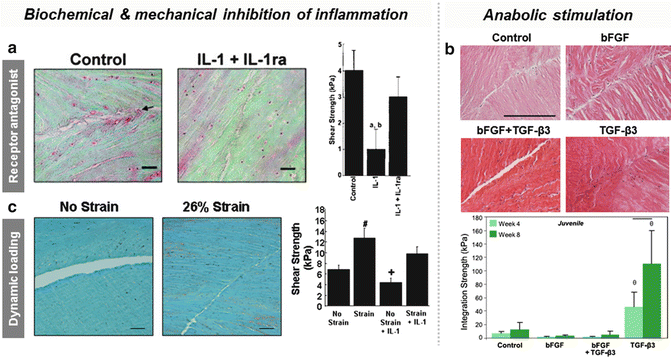
Fig. 10.5
Modification of the environment via biochemical and mechanical cues can augment integration. (a) The addition of IL-1 receptor antagonist (IL-1ra) in the presence of inflammatory cytokine IL-1 increases integrative shear strength of porcine meniscus in vitro (reprinted with permission from John Wiley and Sons from reference [32]). (b) Supplementation with the pro-matrix growth factor TGF-β3 improves integration strength of juvenile bovine meniscus in vitro, while addition of promitotic bFGF does not enhance repair, even when applied in conjunction with TGF-β3 [98]. (c) The application of dynamic loading increases integrative shear strength of porcine meniscus in vitro, overriding the catabolic effects of IL-1 (reprinted with permission from Elsevier from reference [34])
Methods to Enhance Vascularity in Meniscus Repair
Inability of the avascular, inner portion of the meniscus to heal has provided the impetus to investigate means to enhance vascularity via surgical approaches (Fig. 10.3). These approaches include mechanical rasping, trephination, and the introduction of vascularized grafts to the wound site. Partial removal of the wound interface by abrasion has been shown to encourage vascularization by inducing production and release of growth factors and cytokines beneficial to healing [36, 37]. This technique has been used experimentally to repair longitudinal meniscal tears of the avascular zone in rabbits and humans, although it was reported that success was dependent on the distance of the joint capsule to the tear site as well as the size and stability of the tear, indicating that proximity of a vascularized source was still necessary [38]. This problem can be addressed by creating vascular “access” channels that connect the inner zone to the peripheral vasculature (Fig. 10.3b). The provision of such a vascular conduit was pioneered separately by Gershuni et al. [39] and Zhang et al. [40, 41] in animal models. However, trephined channels are prone to collapse during normal physiologic loading, and the removal of meniscal cores can disrupt the aligned collagen fibers and further destabilize meniscal architecture. In a prospective study, Zhang et al. found that symptomatic re-tear rates decreased after combining arthroscopic trephination with suturing to ensure stability, but healing was not evaluated [42]. To maintain biomechanical integrity after core removal, Cook et al. developed a bioabsorbable conduit composed of poly(l-lactic) acid that allowed for fibrovascular tissue ingrowth, which proved superior to trephination alone in a canine model [43] (Fig. 10.3c). Alternatively, parameniscal synovial abrasion can be applied to increase vascularity and provide chemotactic factors up to 5 mm from the outer meniscal rim [44]. Vascularized synovial flaps can also be placed within the meniscal defect to provide both a blood supply and a source of synoviocytes that can infiltrate the wound site and lay down matrix to bridge the gap [19, 39, 45, 46] (Fig. 10.3d). Indeed, interpositional free synovial autografts can improve integration in vitro despite these grafts lacking connections to the systemic vasculature in this model. This indicates that the mechanism of action may be in provision of an added source of regenerative cells or by the graft itself acting as a scaffold for cell migration [45, 47]. In fact, it is thought that superficial cells of the meniscus originated from the synovium and that these cells may be implicated in meniscal healing [48, 49].
To enhance vascularity without surgically removing or introducing tissue, angiogenic factors such as vascular endothelial growth factor (VEGF) [50, 51] and angiogenin [52] have been utilized to promote neovascularization (new vessel formation) at the site of repair. VEGF is a potent endothelial cell chemokine and mitogen that induces formation of new capillaries when delivered at high concentrations [53], whereas angiogenin interacts with both endothelial and smooth muscle cells to promote formation of vascular structures [54]. Although angiogenin was found to promote neovascularization of the avascular zone in rabbits, two sheep studies investigating the application of VEGF released through poly(d,l-lactide) acid suture coatings concluded that local treatment with VEGF did not enhance meniscal angiogenesis or healing in either the vascular or avascular zone [50, 51] (Fig. 10.3e). In these latter studies, the authors speculated that a longer duration of growth factor delivery, using a combination of several angiogenic factors involved in vessel wall assembly, may be necessary. These issues could be addressed by using a composite scaffold, where discrete components can be incorporated to release multiple bioactive factors with differing temporal release profiles [55]. For instance, Ionescu et al. recently developed an electrospun scaffold for fibrous connective tissue engineering that releases VEGF rapidly from water-soluble poly(ethylene oxide) nanofibers and gradually from embedded poly(lactic-co-glycolic acid) microspheres [56]. Using this versatile platform of gradual elution, a combination of VEGF, angiogenin, platelet-derived growth factor, and/or angiopoietin may be used to promote neovascularization.
Endogenous cells can also be acquired from the vasculature to increase cellularity and stimulate healing at the wound site, either via the introduction of a fibrin clot or through microfracture of the underlying bone. It has been argued that it is the hematoma and subsequent clotting, rather than the continuous bloody supply, that are critical to the induction of a repair response [57]. Acting as a source of reparative cells, growth factors, and temporary matrix, exogenous fibrin clots can be precipitated and inserted into meniscal defects to stimulate a healing response [58–62]. In a canine model, Arnockzy et al. found that meniscal defects filled with fibrin clots remodeled into fibrocartilage with chondroid cells after 6 months, although the reparative tissue could still be histologically distinguished from native tissue [58]. Clinical repair of acute, isolated meniscal tears, including complete radial tears that cross avascular regions, were also improved after clot injection [60, 62, 63]. Despite some experimental success, fibrin clots have not been widely adopted because they are difficult to place arthroscopically and are often destroyed or lost during the insertion process. To prevent loss after placement, the fibrin clot can be covered by a fascia sheath [61] or secured by a suture [64] (Fig. 10.3f). A minimally invasive intra-articular method to create a fibrin clot in situ was also developed to bypass the difficulty of clot acquisition and placement [65]. Similarly, an artificial hematoma can be created by releasing marrow elements into the joint space via microfracture of the intercondylar notch [66, 67] to expedite early recovery. To attract and protect endogenous fibrin clots and other host elements after hemarthrosis, small intestine submucosa grafts may be used to promote cellular attachment and expedite regeneration [68–71].
Methods to Improve Cellularity in Meniscus Repair
While strategies for enhancing vascularization are promising, a more established approach is to harvest and deliver therapeutic cells to the wound site, independent of the blood supply (Fig. 10.4). Cells can be recruited from adjacent tissues, injected directly into the lesion, or delivered via a scaffold to improve the intrinsically low cellularity of the native tissue. Ideally, cells should be autologous and abundant, easily acquired and expanded in vitro, and able to produce fibrocartilaginous matrix [72]. The success of autologous chondrocyte implantation (ACI) for cartilage repair demonstrates that cell therapy is clinically feasible and could be adapted to improve meniscus repair. Of particular interest for such applications are synoviocytes, chondrocytes, and meniscal cells, as well as multipotent mesenchymal stem cells (MSCs) that can differentiate into a meniscal-like cell phenotype.
Located just adjacent to the meniscus, fibroblast-like type B synoviocytes produce matrix proteins such as collagen and hyaluronan for the intimal interstitium [73]. Studies by Arnoczky et al. [74] have demonstrated that these cells can cross the synovio-meniscal boundary to reach the wound site and contribute to the repair process. It is also likely that cells of synovial origin play a role in integrating free synovial grafts [45, 47] as well as repopulating meniscal allografts [75, 76]. To that end, parameniscal abrasion of the synovial intima may liberate these cells and expedite their migration to the defect [77]. On the other hand, chondrocytes are typically harvested from articular cartilage, expanded in vitro, and delivered to the lesion site seeded inside a biocompatible scaffold. When inserted into a defect, allogenic chondrocytes improved bonding of the meniscal margins by depositing cartilaginous matrix that integrated the scaffold, in this case devitalized meniscus, with the native tissue [78]. In a more recent integration study, Weinand et al. found that auricular chondrocytes seeded onto Vicryl meshes may provide more healing capacity than articular chondrocytes due to increased production of elastin [79]. Unlike synoviocytes and chondrocytes, meniscal cells have been evaluated more for their use in engineering replacement tissue than for their role in directly modulating repair. Nevertheless, due to their ability to deposit fibrocartilage when seeded onto 3D scaffolds, meniscal cells have potential to be used in the same way as chondrocytes for integrative repair [80–84]. Interestingly, the ability of meniscal cells to produce ECM in vitro does not decrease with age, making meniscal surgical debris a potential source of viable cells for implantation [83].
Another source of regenerative cells for meniscus repair applications is undifferentiated mesenchymal stem cell (MSCs). These cells are capable of differentiating into chondrocytes or cells of other mesodermal lineage if provided the appropriate biochemical and mechanical cues. MSCs can be obtained from several sources, including bone marrow, synovium, and adipose tissue, thus bypassing the need to harvest meniscal or chondral tissue to isolate autologous cells. The most common technique for MSC delivery is injection either into the joint space or directly into the tear site, which is then often closed with sutures (Fig. 10.4a). Abdel-Hamid et al. found that injection of bone marrow MSCs into a meniscal tear in a canine model improved healing by promoting angiogenesis, chondrogenesis, and collagen fiber deposition [85]. In rat and rabbit models, fluorescently labeled synovial-derived MSCs injected into the joint space filled meniscal defects and differentiated into chondrocytes that produced type II collagen [86–88]. Adipose-derived MSCs produced similar regenerative effects when injected directly into avascular meniscal lesions in rabbits [89]. The first human case of autologous MSC transplantation for meniscal cartilage regeneration was done via percutaneous intra-articular injection [90]. Bone marrow-derived MSCs were harvested from the iliac crest, expanded in vitro, and injected into the joint space of an osteoarthritic knee. Analysis via MRI showed evidence of increased meniscal volume after 3 months, although a biopsy was not performed to determine the composition of the new tissue.
Despite the promise of cell therapy, it is often difficult to localize or retain cells at the lesion using injection alone. A study by Agung et al. tracking intra-articular injected bone marrow MSCs with green fluorescent protein (GFP) found that MSCs must be delivered in high numbers (1 × 107) for them to actively mobilize to the meniscus and generate extracellular matrix at the site of injury. However, this high concentration of cells also caused the formation of unwanted scar tissue [91] and may result in the undesirable colonization of surrounding structures. Consequently, it may be more effective to localize cell delivery to the region of interest with the use of a pre-seeded biodegradable scaffold (Fig. 10.4b). For example, fluorescent tracking of transplanted MSCs that were embedded in fibrin glue demonstrated retention of cells inside the meniscal defect [92, 93]. Other successful biomaterials that have been used include collagen scaffolds [94] and hyaluronan-collagen composites [95] (Fig. 10.4c). It is important to note that while pre-culture of cells may generate fibrocartilage and thus improve mechanical properties, formation of new tissue also could inhibit integration of the scaffold in vivo [95, 96]. Thus, while there are still many factors to optimize for cell-based treatments, there is strong evidence that viable autologous cells, and MSCs in particular, can significantly augment meniscal healing.
Influence of Soluble Factors on Meniscus Repair
The presence of viable cells at the wound site does not in and of itself guarantee a robust repair response. To expedite the healing process, cells should be in a noninflammatory, instructive environment that encourages proliferation and synthesis of extracellular matrix. McNulty et al. demonstrated that inhibition of inflammatory cytokines IL-1β and TNFα with IL-1 receptor antagonist (IL-1ra) and anti-TNFα monoclonal antibody (TNFα mAB), respectively, as well as inhibition of broad-spectrum matrix metalloproteinase (MMP) activity, improved the integration strength of meniscal explants in vitro [32, 33] (Fig. 10.5a and Table 10.1). MMP inhibitors, such as tetracycline, hold promise as agents which may potentiate meniscal healing. Conversely, growth factors such as transforming growth factor beta (TGF-β) [97–102], platelet-derived growth factor AB (PDGF-AB) [99, 102–106], insulin-like growth factor 1 (IGF-1) [99, 100, 102, 106, 107], and basic fibroblast growth factor (bFGF) [57, 99, 100, 108, 109] have been investigated for their mitogenic and anabolic effects on meniscal cells (Table 10.1). Since integrative strength is correlated with collagen deposition and cross-linking [110], the most significant improvement occurs when ECM synthesis increases as a result of growth factor stimulation. Throughout the literature, the most successful growth factor for increasing cell division and proteoglycan and collagen production in cell cultures and tissue explants in vitro is TGF-β1 [99]. McNulty et al. found that TGF-β1 supplementation increases the interfacial shear strength of meniscal explants enough to overcome the catabolic effects of IL-1-mediated MMP activity [101]. TGF-β3 has also been investigated for its pro-matrix effects and has been shown to improve the integration of juvenile and adult meniscal explants when delivered continuously over time in vitro [98] (Fig. 10.5b). Growth factors of intermediate efficacy include PDGF-AB and IGF-1 [99]. PDGF-AB also induces meniscal cell migration [106] as well as proteoglycan production [99, 105], making it an attractive agent for recruiting endogenous cells to the wound margin. However, a region-specific response to PDGF-AB has been reported, where proliferation increased within meniscal explants from the peripheral zone but not so in the central region, possibly due to local variation in growth factor receptors [103]. Subsequent studies found that meniscal cells from all zones show a proliferative response in monolayer culture, suggesting that regional matrix sequestration of soluble factors may be the cause instead [104, 106]. IGF-1 supplementation similarly enhances cellularity and ECM synthesis and is especially stimulatory for cells in the inner avascular zone [107]. Less successful have been growth factors that stimulate proliferation but little to no increase in matrix protein production, such as bFGF [57, 98–100, 102, 108], perhaps because less resources are devoted to repair when cells are in a mitotic state (Fig. 10.5b). In this case, it may be beneficial to deliver a mitogen over the short term, coupled with sustained delivery of a pro-matrix growth factor either via drug-releasing scaffolds or sutures, or gene transfer to ensure long-term stability.
Table 10.1
Biologic factors of interest for meniscal repair and their effect on meniscal cell behavior and impact on integrative potential
Growth factor | Cellular effect | Integrative potential | Methods |
---|---|---|---|
TGF-β | Potent stimulator of matrix deposition | Increased | |
PDGF-AB | Intermediate stimulator of matrix deposition, proliferation, and migration | No data | |
IGF-1 | |||
bFGF | Potent stimulator of proliferation | No effect | ![]() Stay updated, free articles. Join our Telegram channel![]() Full access? Get Clinical Tree![]() ![]() ![]() |