Chapter 26 Articular Cartilage Repair With Bioscaffolds
The goal of articular cartilage restoration is to recreate normal hyaline cartilage at the site of a cartilage defect. Currently, this has only been achieved through osteochondral transfer, noting that limitations of autograft and allograft implants preclude widespread use. Although hyaline-like tissue properties may be demonstrated using cell therapy alone, the tissue lacks the natural stratification of normal hyaline cartilage. As with most bodily tissues, the natural structure serves a distinct purpose—efficiency of resources and energy, function (low coefficient of friction, dispersal of loads to underlying bone both spatially and temporally), and durability. If the goal is to restore these articular cartilage attributes, all aspects of normal hyaline cartilage must be addressed: basilar integration with bone and calcified cartilage, marginal integration, filling of the defect level to the surrounding normal cartilage walls, and natural stratification duplicating the variability of the morphology and density of chondrocytes, as well as the regional differences in the extracellular matrix. To achieve these goals, one approach uses a scaffold to influence the cells. There are many variations on this theme, ranging from the most basic scaffold, the fibrin clot that occurs with any marrow stimulation procedure, to three-dimensional multiphasic (osteochondral promoting) scaffolds, with chondrocytes seeded and cultured in a stratified manner.5,18,29,41
Part I: Scientific Basis for Design Considerations of Bioscaffolds
Scaffold Requirements
The second role, to act as a support structure during cell and ECM maturation, is far more complex and may require more advanced engineering to produce a scaffold that optimizes the physical and biochemical structure of the ECM while providing adequate porosity to allow cell invasion and growth (Table 26-1).27 The ideal scaffold should allow early or even immediate weight bearing; thus, it would require mechanical properties strong enough to protect the cells while at the same time not being so stiff as to completely shield the cells from all stresses, which are important signals for tissue maturation. The scaffold should provide secure fixation and enhance basilar integration with the subchondral bone and circumferential integration with the surrounding cartilage. To allow cell growth, the scaffold must consist of a system of interconnected pores; the material should be hydrophilic to ease cell seeding, penetration, and adhesion. Furthermore, certain modifications can improve cell adhesion to a scaffold, such as binding of adhesion ligands to the scaffold material. It should slowly resorb with time, at a pace that allows gradual replacement through host tissue, and this process should not generate degradation products that are toxic or inflammatory.
Table 26-1 Desirable Attributes for Bioscaffolds Used in Cartilage Repair
Structure and Chemistry | Mechanical Properties, Strength, and Integrity | Clinical Application |
---|---|---|
Biocompatible synthetic versus naturally derived | Mechanical properties comparable to hyaline cartilage | Preformed intraoperatively versus custom shape or contour versus injectable |
Porosity-permeability—optimal porosity with three-dimensional architecture | High porosity (does not apply to gel-type scaffolds) | Ease of intraoperative handling and fixation |
Optimized geometry to regenerate native matrix (ECM) orientation | Composite structure with varying properties throughout its thickness | Delivery attributes—arthroscopic (air or liquid) versus miniopen versus formal open |
Resorption without local or systemic adverse effect versus benign particulate breakdown scavenging | Biocompatible | Chondral versus osteochondral defects |
Resorption temporal profile follows new cartilage deposition | No toxic degradation products | Reproducibility |
Surface chemistry (protein absorption-deposition, enabling cell adhesion, migration, and outgrowth) | Assists in cell and tissue differentiationResorbable | Regulatory approval pathway and final indicationsCost, value |
Basic Science
Physical Characteristics
Mechanical Strength
Mechanical characteristics of hyaline cartilage vary with the joint in question, as well as the specific location within the respective joint. A bioscaffold allowing early or even immediate weight bearing is desirable and should closely mirror the mechanical properties of hyaline cartilage until it has been replaced by mature repair tissue. The scaffold functions to protect the growing tissue while ensuring an appropriate level of physiologic loading to enhance the reparative process,47 an effect first described by Pauwels,48 who recognized the influence of physical stimuli on cell differentiation pathways of mesenchymal stem cells.
Elasticity (Young’s modulus) of human hyaline cartilage has been reported as between 1 and 20 Mpa, depending on the layer and location, several orders of magnitude lower than that of immature (1000 MPa) or cortical (17,000 MPa) bone.34 It appears from computer modeling that an inhomogeneous three-dimensional scaffold with higher stiffness in the superficial layer, which gradually decreases toward the base of the defect, might be best suited to encourage cartilage, rather than fibrous tissue, regeneration. This theory has been substantiated by findings of a tensile modulus 6 to 20 times higher in the superficial regions than in the deeper regions, whereas permeability demonstrated a reverse distribution, increasing with increasing depth. The higher stiffness at the surface better protects the immature tissue from the high shear forces experienced at this level and the lower stiffness at the base allows sufficient strain rates to encourage chondrogenic differentiation.
Structure
Studies have investigated effects of the overall three-dimensional structure of scaffolds on cells and tissue production. Although chondrocytes attach and grow even on flat nonphysiologic surfaces (two-dimensional growth, such as in a Petri dish), they gradually dedifferentiate into a more fibroblastic phenotype with increased type I collagen production. Conversely, chondrocytes maintain their spherical appearance when grown in three-dimensional culture, such as open-pored scaffolds or alginate beads, and matrix production is improved quantitatively and qualitatively with increased type II collagen.41 Cartilage ECM consists of a mesh of collagen fibers 10 to 140 nm in diameter53 and studies have demonstrated improved cell adherence to fibers of submicron size.65 Many studies have therefore investigated the use of spun or woven nanofibers of various materials for use in bioscaffolds.26
A system of interconnected open pores facilitates cell seeding of bioscaffolds to produce a three-dimensional structure. The normal pore area of hyaline cartilage has been reported as 5 to 33 nm,52 but this is not directly comparable to the requirements of a bioscaffold. The former reflects the size of a lacuna, but pores in a bioscaffold have to be large enough to allow cell seeding, penetration, and proliferation, followed by production of ECM. However, increased pore size beyond a threshold value has been demonstrated to decrease attachment for a variety of cells, whereas increased specific surface area (a measure of overall porosity) was found to have a positive effect.45 In general terms, a material porosity of 80% to 90% has been found to be beneficial in terms of quantity and quality of regenerated tissue.28 In addition to the pore size, which allows cell migration, the nanostructure of the material must allow cell adherence during migration.
Biologic Characteristics
Biocompatibility
More commonly an issue with synthetic bioscaffolds, biocompatibility refers to tissue reactivity toward the implanted material. Biocompatibility can be improved by surface modification of the material to improve cell adhesion—for example, the wettability of hydrophobic polymers, such as the polyesters PGA and PLA, can be improved by gas plasma treatment to polymerize specific monomers to the scaffold surface.46 Biomolecules can also be attached, such as arginine-glycine-aspartic acid (RGD), which interact with integrin receptors to anchor the cell cytoskeleton to the ECM.57 However, even within the group of biologic scaffold materials, such as collagen and hyaluronic acid, subtle variations exist that influence cell adhesion. In a review of several collagen membranes, type II collagen appeared to be better suited to enhance cell attachment than type I collagen membranes.23,36
Degradation
Generally a concern with artificial scaffold materials, degradation products seen during absorption can lead to foreign body reactions and inflammatory responses. For example, both PGA and PLA degradation through hydrolytic cleavage of ester bonds can result in acidic byproducts62 that have been implicated in foreign body and other inflammatory reactions. Buffer substances can be added to influence the rate of resorption as well as the acidity of degradation products.
Bioactivity
Ideally, a bioscaffold will provide not only mechanical support but guide the cells contained within to produce a better repair tissue. Attaching growth factors to the scaffold material has been investigated by several authors, who reported a shift to a more hyaline-like appearance of regenerated cartilage after the addition of various factors, including the bone morphogenic protein (BMP), insulin-like growth factor (IGF), and transforming growth factor (TGF) families.2
Part II: Scaffolds in Development
Part I of this chapter established the scientific basis for the use of scaffolds in the repair of articular cartilage defects of the knee. However, at the time of this writing, none are clinically available in the United States. Although the list of desirable attributes for an articular cartilage repair scaffold represents realistic goals, attempting to achieve all the attributes in one scaffold remains elusive.59 As with all aspects of medicine, if there were a true best method or best scaffold, then all physicians would adopt that single technique. However, in this relatively new field, the reality is that many approaches remain under evaluation,11 because none has provided the stated end goal: to produce a true stratified hyaline cartilage, with full basilar and marginal integration implanted, using a minimally invasive technique with minimal inconvenience to the patient and cost to society. Nevertheless, from the view point of demand matching, the laudable but possibly unobtainable stated goal may not be necessary for many knee lesions. Consider that many first-generation cartilage repair techniques appear to work satisfactorily in up to 70% of patients. Therefore, the goal may need to be restated from a patient function and pain perspective, and not from a histologic perspective. That is, the cartilage repair goal may be the most cost-effective and acceptably durable treatment for a specific patient and specific cartilage lesion, rather than a fully integrated hyaline cartilage. This on the ground clinical approach should not deter basic science research, but illustrates the difference between preclinical results and clinical applications. It is important to keep the patient’s knee in mind while exploring the newer scaffold cartilage repair options.43
The first clinical application of a scaffold for knee cartilage repair was reported in 1998 by Behrens.8 The two-stage technique was an extension of the original ACI. After an autologous biopsy was cultured, the chondrocytes were seeded onto a porcine collagen I-III scaffold (Chondro-Gide, Geistlich Biomaterials, Wolhusen, Switzerland) and allowed to grow on the scaffold before implantation. It was termed matrix-associated autologous chondrocyte implantation (MACI; Genzyme, Cambridge, Mass). Shortly thereafter, in 1999, Hyalograft C was introduced. The scaffold was a benzylic ester of hyaluronic acid (HYAFF 11, Fidia Advanced Biopolymers Laboratories, Padova, Italy).69 Like the two-stage MACI, the autologous cartilage was harvested from the patient, followed by expansion and seeding onto the scaffold, where they are allowed to grow. Both of these three-dimensional scaffolds have been shown to improve the maintenance of a chondrocyte-differentiated phenotype when compared with two-dimensional culturing. These initial biodegradable polymers remain in active clinical use in Europe, with many reports of efficacy over time, and have been joined by several other seeded, cultured scaffold applications. In addition, these scaffolds allowed arthroscopic implantation in certain regions of the knee, typically the femoral condyles and trochlea, which was not possible with first-generation ACI. Because this is a rapidly changing field, our goal here is to show current scaffold applications in a general sense, with the understanding that the initiated reader will review current literature and conference presentations before making any clinical decisions. In addition, it is necessary for the reader to fully understand the regulatory process in his or her respective country because allowed clinical use may vary over time.
Scaffolds Without Cells at Time of Implantation (Host Cell Source)
Autologous Matrix-Induced Chondrogenesis
Autologous matrix-induced chondrogenesis (AMIC) is a porcine collagen I-III matrix (Chondro-Gide) that is applied over the defect immediately following microfracture. This scaffold is thicker than the original one used in MACI to allow potential filling of a full bottom to top defect. The goal is to provide a matrix that allows host cells to migrate into the scaffold and have an environment that improves the chondrogenesis from that of marrow stimulation alone.22,31
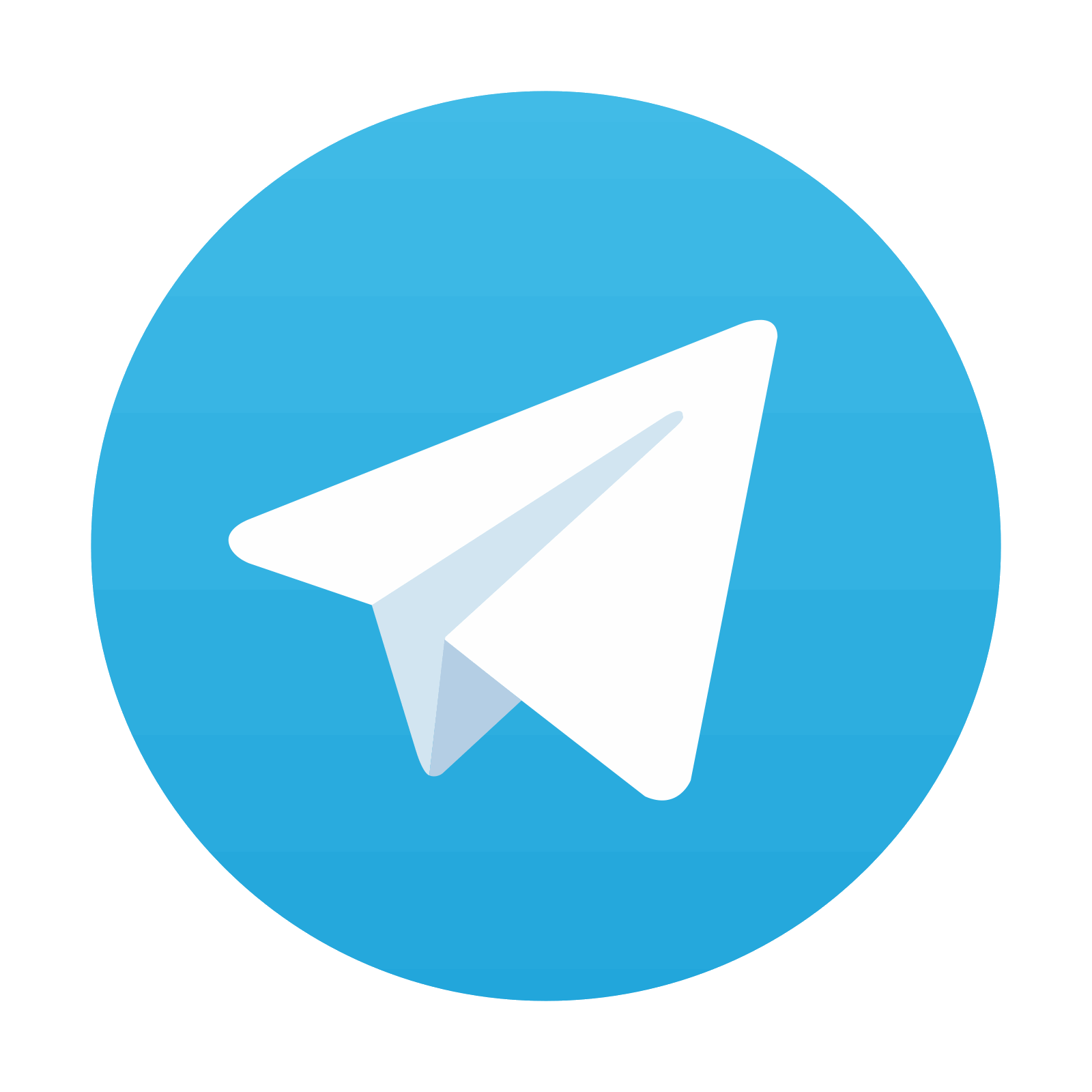
Stay updated, free articles. Join our Telegram channel
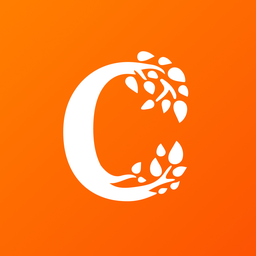
Full access? Get Clinical Tree
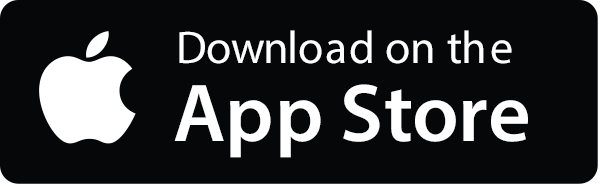
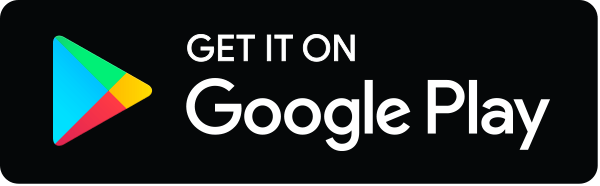