Aquatic Rehabilitation
Bruce E. Becker
Andrew J. Cole
INTRODUCTION AND BRIEF HISTORY
Historical Applications
Throughout all recorded history, the sick and suffering have resorted to springs, baths, and pools for their soothing, healing, and powerful effects. “Taking the waters,” soaking in baths and pools, and resting at places called spas played an important social and spiritual role in the river valley civilizations of Mesopotamia, Egypt, India, and China. Ritual bathing pools were widely used for individual and social renewal and healing. Healing water rituals also appeared in ancient Greek, Hebrew, Roman, Christian, and Islamic cultures (1). During the Middle Ages in Europe, the beginnings of formal resorts formed for the purposes of healing began to emerge. These became the progenitors of the current group of European spas. Dr. Sidney Licht (2) a physiatrist and founding member of the American Society of Medical Hydrology and Climatology (ASMH), defined a spa as a “place where mineral containing waters flow from the ground naturally, or to which it is pumped or conducted, and is there used for therapeutic purposes.”
In the decades before the American Civil War, major health reforms swept the nation, and numerous hydropathic establishments, institutes, and medical colleges were built for the practice of water cure cold water bathing. The American Medical Association Committee on Sanitaria and Springs published its first national report on sanitaria in 1880. At the turn of the century, medical men such as Simon Baruch, John Harvey Kellogg, and Guy Hinsdale regularly conducted clinical hydrotherapeutic experiments and prescribed aquatic therapeutics. Baruch established the American medical standards for hydrotherapy in The Principles and Practice of Hydrotherapy, and in 1921, shortly before he died, Baruch published his last book, An Epitome of Hydrotherapy (3,4). Baruch writes in the Preface to the 2nd edition of The Principles and Practice of Hydrotherapy, “So flexible is this therapeutic agent that, unlike medicinal remedies, it may be utilized to meet indications which seem contradictory to the uninitiated” (3).This statement holds true today.
Although most writings concerned the internal and external curative benefits of the waters, baths, and pools, limited emphasis was placed on water exercise. In 1911, Charles Leroy Lowman began using therapeutic tubs to treat spastic patients and those with cerebral palsy. Lowman founded the Orthopaedic Hospital in Los Angeles in 1913, later to become Rancho Los Amigos. He visited the Spaulding School for Crippled Children in Chicago, where he observed paralyzed patients exercising in a wooden tank. On his return to California, he transformed the hospital’s lily pond into two therapeutic pools (3,4). He also made a motion picture showing the various types of cases treated with pool therapy. At Warm Springs, Georgia, Leroy Hubbard developed his famous tank, and in 1924, Warm Springs received its most famous aquatic patient, Franklin D. Roosevelt.
At Saratoga Springs, New York, financier Bernard M. Baruch, Roosevelt’s friend and the son of Dr. Simon Baruch, headed a special commission to plan a scientific American spa (5). The commission studied spa design, natural treatments, and efficient operations based on what was then felt to be the sound medical and scientific care for chronically ill patients, especially those suffering from cardiac, vascular, and circulatory ailments, and selected Dr. Walter S. McClellan to be the Medical Spa Director (6). Drs. W. E. Fitch and McClellan, and several other spa doctors, scientific directors, and spa general managers met at French Lick Spa to organize “an association which would be of mutual benefit to everyone interested in the advancement of (Medical) Hydrology in this country” (7). A wealth of information, research, and articles on health resort medicine, spa therapy, and pool treatments appeared in professional journals during the 1930s. At Northwestern University Medical School in Chicago, Dr. John S. Coulter (8) presented lectures on physical therapy that he placed within the history of spa medicine. In 1933, the Simon Baruch Research Institute of Balneology at Saratoga Springs Spa was established, and the facility began the printing of their scientific bulletin, The Publications of Saratoga Spa. At Hot Springs, Arkansas, a warm swimming pool was installed for special underwater physical therapy exercises and pool therapy treatments for chronic arthritic patients (9). By 1937, Dr. Charles Leroy Lowman published his Technique of Underwater Gymnastics: A Study in Practical Application, in which he detailed pool therapy methods of specific underwater exercises that “carefully regulated dosage, character, frequency, and duration for remedying bodily deformities and restoring muscle function” (10). During the 1950s, the National Foundation for Infantile Paralysis supported the corrective swimming pools and hydrogymnastics of Charles L. Lowman and the therapeutic use of pools and tanks for the treatment of poliomyelitis. In 1962, Drs. Sidney Licht, Herman Flax, Sigmund Foster, William Erdman, Lucille Eising, J. Wayne McFarland, Jens Henriksen, and Richard Gubner organized the ASMH, which traditionally continues to meet at the annual meeting of the American Academy of Physical Medicine and Rehabilitation.
Current Trends and Uses
With the end of the polio epidemic and the rise of newer and more exciting technology in rehabilitative therapeutics, the use of the aquatic environment in rehabilitation waned. Medical hydrology became a smaller and smaller component of physiatric and physical therapy training. Principles of medical hydrology and aquatic rehabilitation that were formerly an important part of a therapist’s training program were deemphasized. Since the mid-1980s, the increase in health-care costs, constraints on physical therapy utilization under managed care, and an emerging public understanding of the value of aquatic rehabilitation and better training within the rehabilitative professions have begun to drive a broader utilization of aquatic therapies. Fortunately, basic science research in the biologic effects of immersion accelerated during the late 1960s for two fortuitous reasons. Water was recognized as a wonderful surrogate for the weightlessness of space, and as we prepared to place humans outside gravity, it became essential to forecast the effects of space flight. At the same time, Murray Epstein and other endocrinologists realized that aquatic immersion was a benign means of simulating central volume expansion to better understand volume homeostasis. The end result of these research efforts has been that aquatic therapy has a considerable foundation of high-quality basic science research.
THE PHYSICS OF WATER AND ITS RELATIONSHIP TO AQUATIC REHABILITATION
Density and Specific Gravity
Density is defined as mass per unit volume and is given the Greek letter rho (ρ). The relationship of ρ to mass and volume is characterized by the following formula:
ρ = m/V
where m is the mass of a substance whose volume is V. Density is measured in the international system by kg/m3 and occasionally as g/cm3. Density is a temperature-dependent variable, although much less so for solids and liquids than for gases. In addition to density, substances are defined by their specific gravity, the ratio of the density of that substance to the density of water. By definition, water has a specific gravity equal to 1.00 at 4°C. Salt water density varies considerably, from ocean to ocean, up to the Dead Sea, with a density greater than 1.16 (11). (The Black Sea has the lowest ocean density nearly approximating 1, due to significant fresh water inflow, but this has created significant environmental concerns.) Although the human body is mostly water, the body’s density is slightly less than water and averages a specific gravity of 0.974, with males averaging higher density than females. Lean body mass, which includes bone, muscle, connective tissue, and organs, has a typical density near 1.10, whereas fat mass, which includes both essential body fat plus fat in excess of essential needs, has a density of about 0.90 (12). Consequently, the human body displaces a volume of water weighing slightly more than the body, forcing the body upward by a force equal to the volume of the water displaced. The buoyancy differences between lean and fat body mass make aquatic activity particularly useful for severely obese individuals, for whom land-based exercise may produce joint pain.
Hydrostatic Pressure
Pressure is defined as force per unit area, where the force F by convention is understood to act perpendicularly to the surface area A. This relationship is
P = F/A
The standard international unit of pressure is called a pascal (Pa), after the French scientist Blaise Pascal, and is measured in Newtons (N)/m2, dynes/cm2, kg/m2, and pounds per square inch (PSI). Fluids have been experimentally found to exert pressure in all directions. The pressure exerted on a theoretical point surrounded by a fluid is equal from all directions. Obviously, if unequal pressure were being exerted, the point would move until the pressures were equalized on it. Pressure is directly proportional to both the liquid density and the immersion depth, when the fluid is incompressible, as water is at the depths used in therapeutic environments. Because pressure responds not only to the fluid depth but also to any force exerted on its surface, the pressure of the Earth’s atmosphere is an important contributor to the total force from immersion. Water exerts a pressure of 22.4 mm Hg for every foot of water depth, which translates to 0.73 mm Hg/cm or slightly under 2 mm Hg/in. of H2O depth. Thus, a body immersed to a depth of 48 in. is subjected to a distal pressure equal to 88.9 mm Hg, far greater than venous or lymphatic pressures. This external compressive force significantly aids the resolution of edema in an injured body part or where postsurgical edema resolution is a therapeutic goal.
Buoyancy
Buoyancy causes immersed objects to have less apparent weight than the same object on land. Buoyancy, a force opposite to gravity, acts on the object with a force generated by the volume of H2O displaced. This principle was discovered by Archimedes (287 to 212 B.C.) and is the reason that water can be used to advantage in the management of medical problems, such as arthritis or severe obesity requiring weight off-loading. A human with a specific gravity of 0.97 will reach floating equilibrium when 97% of his or her volume is submerged.
Because the force of buoyancy is an upward force, there are important consequences in the therapeutic aquatic environment. The center of gravity is a point at which all force moments are in equilibrium. For an average male adult human being standing in the “anatomic” position, this point is located slightly posterior to the midsagittal plane and at about the level of the second sacral vertebra, because the human body is nonuniform with respect to density and varies depending upon body build within a radius of 1.9 to 9 cm (13). The lungs
obviously are less dense than the lower limbs, for example. The center of buoyancy is defined as the center of all buoyancy force moments summating on each body segment. Typically, the human center of buoyancy is in the midchest. The difference between the center of gravity (a downward force) and the center of buoyancy (an upward force) may generate rotational torque. This rotational torque may produce instability for some patients and should be carefully monitored, preferably by a therapist in the pool with the patient.
obviously are less dense than the lower limbs, for example. The center of buoyancy is defined as the center of all buoyancy force moments summating on each body segment. Typically, the human center of buoyancy is in the midchest. The difference between the center of gravity (a downward force) and the center of buoyancy (an upward force) may generate rotational torque. This rotational torque may produce instability for some patients and should be carefully monitored, preferably by a therapist in the pool with the patient.
Water in Motion
Flow Characteristics
When water moves smoothly, in layers moving at the same speed, the water is defined as in laminar or streamline flow. When water moves more rapidly, even minor oscillations create uneven flow, and parallel paths are knocked out of alignment, resulting in turbulent flow. Within the mass of water, flow patterns arise that run dramatically out of parallel. These paths are called eddy currents. An example of the latter are the eddy currents that form in the blood stream behind artery walls encrusted with cholesterol plaque. Turbulent flow absorbs energy at a much greater rate than streamline flow, and the internal friction within the fluid determines the rate of energy absorption. This internal friction is called viscosity. The major determinants of water motion are viscosity, turbulence, and speed. Flow rates decrease when turbulence occurs, largely due to the significant nonlinear increase in internal friction in the fluid. The onset of turbulent flow obviously is a function of fluid velocity, but it is also related to fluid density, viscosity, and enclosure radius. The transition from laminar flow to turbulent flow often occurs abruptly with increasing velocity.
Viscosity and Drag
Viscosity refers to the magnitude of internal friction specific to the fluid. As layers of fluid molecules are set into motion, molecular attraction creates resistance to movement and is detected as friction. Energy must be exerted to create movement, and as in the first law of thermodynamics, energy is never lost but rather is transformed and stored as potential or kinetic energy. Some energy is transformed into heat, some into kinetic energy, and some may be stored as potential energy by increasing surface tension. Fluids are in part defined by individual viscosity, expressed quantitatively as the coefficient of viscosity (Table 62-1). The greater the coefficient, the more viscous the fluid and the greater the force required to create movement within the fluid. This force is proportionate to the number of molecules of fluid set into motion and the velocity of movement. The SI unit of measurement of viscosity is called a poise, after the French scientist J. L. Poiselle (1799 to 1869), who studied the physics of blood circulation. Because velocity is described by distance/time, viscosity is the first time-dependent property. An equation that expresses this relationship must define the volume of the fluid in motion, measured as the area (A), depth (l), and velocity (v) of the motion.

TABLE 62.1 Coefficients of Viscosities for a Variety of Fluids | |||||||||||||||||||||
---|---|---|---|---|---|---|---|---|---|---|---|---|---|---|---|---|---|---|---|---|---|
|
Water is intermediate in viscosity as liquids go, but it still presents much resistance to movement. Calculation of these forces has been done and a mathematical model has been developed using a prosthetic leg model (14). Under turbulent flow conditions, this resistance increases as a log function of velocity. The greatest surface area drag on a swimming man is his head, although the negative pressure following the swimmer causes the greatest force resisting forward movement. There is turbulence produced by fast-moving body surface areas and a drag force produced by the turbulence behind. Viscosity, with all its attendant physical properties, is a quality that makes water a useful strengthening medium. Viscous resistance increases as more force is exerted against it, but resistance drops to zero almost immediately on cessation of force because there is only a small amount of inertia (viscosity effectively counteracts inertial momentum). Thus, when a rehabilitating person feels pain and stops movement, the force decreases precipitously, and water viscosity damps movement almost instantaneously. This allows great control of strengthening activities within the envelope of patient movement comfort.
Specific Heat
Water is used therapeutically in all three of its thermal states: solid, liquid, and gas. A major reason for its usefulness lies in the physics of aquatic thermodynamics. All substances on earth possess energy stored as heat. This energy is measured in a quantity called a calorie (cal). A calorie is defined as the heat required to raise the temperature of 1 g of water by 1°C (e.g., from 14.5°C to 15.5°C). The energy required to raise temperature is defined in kilocalories, the amount of energy required to raise 1 kg of water 1°C, and this unit by convention is termed a Calorie (with a capital C) (Cal). This is the unit in which food energy content is measured. The British system measures heat energy in British Thermal Units (BTU), the amount of energy required to raise 1 lb of water 1°F (1 BTU = 0.252 Cal). A mass of water possesses a measurable amount of stored energy in the form of heat. Energy stored may be released through a change to a lower temperature, or additional energy may raise the water temperature. The formula defining the quantity of energy required or released is
Q = mcΔT°
where m equals the mass of water, c equals the specific heat capacity of the fluid, and ΔT° equals the change in temperature. The work required to produce this energy is called the mechanical equivalent of heat and is measured in joules (J). One Calorie is equivalent to 4.18 × 103 J. A body immersed in a mass of water becomes a dynamic system. If the temperature of the water exceeds the temperature of the submersed body, the system equilibrates to a different level, with the submersed body warming through transference of heat energy from the water, and the water cooling through loss of heat energy to the body. By the first law of thermodynamics, the total heat (and thus energy) content of the system remains the same. Energy applied to this system increases the kinetic energy of some of the molecules, and when high kinetic energy molecules collide with lower kinetic energy molecules, they transfer some of their energy, increasing and equilibrating the total energy of the system. Water is defined as having a specific heat capacity equal to 1.00. Air, in contrast, has a far lower specific heat capacity = 0.001. Thus, water retains heat 1,000 times more than an equal volume of air.
Thermal Energy Transfer
The therapeutic utility of water is greatly dependent on both its ability to retain heat and its ability to transfer heat energy. Exchange of energy in the form of heat occurs in three ways: conduction, convection, and radiation. Conduction may be thought of as occurring through molecular collisions that take place over a small distance. For example, a hydrocollator pack transfers heat by conduction. Substances vary widely in their ability to conduct heat. Convection requires the mass movement of large numbers of molecules over a large distance (i.e., fluid flow). For example, a whirlpool bath transfers heat by convection. Heat transfer across a gradient is measured by the amount of heat in calories transferred per second across an imaginary membrane. Liquids and gases are generally poor conductors but good convectors. Water is an efficient conductor of heat and transfers heat 25 times faster than air. Radiation transfers heat through the transmission of electromagnetic waves. For example, a heating lamp transfers heat by radiation. The rate of radiant energy transfer from a body is proportional to the fourth power of its temperature in degrees Kelvin. It is also proportional to surface area, to the emissivity of the material, and to the second power of the distance between the energy-radiating and energy-absorbing bodies. The thermal conductive property of water, in combination with water’s high specific heat, makes its use in rehabilitation versatile, as it retains heat or cold while transferring it easily to the immersed body part.
BIOLOGIC ASPECTS OF AQUATIC REHABILITATION
Circulatory System Effects
Water begins to exert external pressure on the body immediately on immersion. Intrinsic pressure within the venous and lymphatic side of the circulation is much lower than pressure on the arterial side of the system. Venous and lymphatic pressures vary, depending on the part of the body and its vertical relationship to the heart, but are in part controlled by the system of valves within both systems, which prevents backflow. These one-way valves act to divide the large vertical column fluid into many short columns with little vertical height. This allows much lower hydrostatic pressure gradients inside the vessel wall, so that the maximum venous pressure is 30 mm Hg peripherally, decreasing steadily as blood travels toward the right atrium, which has a negative pressure of -2 to -4 mm Hg. The role of these valves in maintaining a low-pressure system is critical, as can be observed when they fail, creating venous varicosities due to the lack of sufficient vessel wall strength to support the increased height of the fluid column. Consequently, venous and lymphatic returns are sensitive to external pressure changes, including compression from surrounding muscles and from external water pressure. During water immersion, hydrostatic pressure displaces blood upward through this one-way system, first into the thighs, then into the abdominal cavity vessels, and finally into the great vessels of the chest cavity and into the heart. Central venous pressure begins to increase with immersion to the xiphoid and increases until the body is completely immersed. Right atrial distension occurs, and pressure increases by 14 to 18 mm Hg during immersion to the neck, going from about -2 to -4 mm Hg to +14 to +17 mm Hg (15,16). The transmural pressure gradient of the right atrium increases significantly, measured by Arborelius and others at 13 mm Hg, going from 2 to 15 mm Hg. Extra systoles may result, especially early into immersion (15).
Pulmonary blood flow increases with increased central blood volume and pressure. Mean pulmonary artery wedge pressure increases from 5 mm Hg on land to 22 mm Hg during immersion to the neck (16). Most of the increased pulmonary blood volume is distributed in the larger vessels of the pulmonary vascular bed and only a small percentage (5% or less) at the capillary level. This is validated by the fact that the diffusion capacity of the lungs changes very little.
Central blood volume increased by 0.7 L in Arborelius’ classic study (15). This represents a 60% increase in central volume, with one third of this volume taken up by the heart and the remainder by the great vessels of the lungs. Cardiac volume increases 27% to 30% with immersion to the neck (17), but the heart is not a static receptacle. The healthy cardiac response to increased volume (stretch) is to increase the force of contraction. As the myocardium stretches, an improved actin/myosin filament relationship is produced, enhancing the myocardial efficiency (Starling’s law) (18). Mean stroke volume increases 35% on average with immersion to the neck from a resting baseline of about 71 mL/beat to about 100 mL/beat, which is close to the exercise maximum for a sedentary, deconditioned individual on land (19,20). There is both an increase in end-diastolic volume and a decrease in end-systolic volume (15). Stroke volume is one of the major determinants of the increase in cardiac output seen with training because heart rate response ranges remain relatively fixed (19).
Most of the changes are temperature dependent, with cardiac output increasing progressively with increasing water
temperatures. Weston found cardiac output to increase by 30% at 33°C rising to 121% at 39°C (17). There is considerable individual variance in the many studies assessing this phenomenon.
temperatures. Weston found cardiac output to increase by 30% at 33°C rising to 121% at 39°C (17). There is considerable individual variance in the many studies assessing this phenomenon.
As cardiac filling and stroke volume increase with deeper immersion from symphysis to xiphoid, the heart rate typically decreases (16,21). This decrease is variable, with the amount of decrease dependent on water temperature. Typically, at average pool temperatures, the rate decreases by 12% to 15% (17). There is a significant relationship between water temperature and heart rate. At 25°C, the heart rate decreases by approximately 12 to 15 beats/minute (17,22), whereas at thermoneutral temperatures, the rate of decrease is less than 15%, and in warm water, the rate generally increases significantly, contributing to the major increase in cardiac output at high temperatures. The reduction variability is believed to be related to decreased peripheral resistance at higher temperatures and increased vagal effects (23). Ongoing work in our lab, has corroborated the heart rate effects, with healthy college-aged students showing a reduction of heart rate in cool (30°C) water, an increase to about baseline in neutral (36°C), and elevation averaging about 10% in warm water (39°C). (Becker BE, Hildenbrand K, Sanders JP, et al. Biophysiologic Effects of Warm Water Immersion. International Journal of Aquatic Research & Education. 2009;3(1):24-37.) At the same time, by monitoring autonomic nervous system activity, we found elevation of sympathetic activity in cool water, substantially reducing in warm water, with vagal power increasing through the cool to warm immersion sequence. (Becker BE, Hildenbrand K, Sanders JP, et al. Age-Dependent Autonomic Changes Following Immersion in Cool, Neutral, and Warm Water Temperatures. International Journal of Aquatic Research and Education. 2010;4(2):127-146.)
The most efficient way for the heart to deliver more blood during exercise is to increase stroke volume. Maximal myocardial oxygen consumption efficiency (peak heart muscle efficiency) occurs when stroke volume increases because heart rate acceleration is a less efficient means of increasing output (18,24). Thus, as cardiovascular conditioning occurs, cardiac output increases are achieved through smaller increases in heart rate but greater stroke volumes per beat. This is the reason that conditioned athletes are able to maintain lower pulse rates for a given cardiac output than those who are deconditioned (18,24).
Several studies have validated the use of aquatic environments in cardiovascular rehabilitation after infarct and ischemic cardiomyopathy by actively rehabilitating heart patients in an aquatic environment (25). Tei and Tanaka found that a single brief immersion in a hot water (41°C) bath decreased both pulmonary wedge pressure and right atrial pressure by nearly 30%. Over a period of 1 month of daily therapy, patients showed nearly a 30% increase in ejection fraction, significantly improving by one and sometimes two New York Heart Association classifications (26, 27, 28, 29, 30). Recent work on the use of aquatic exercise in heart disease has shown significant benefit in both mild to moderate heart failure and in general cardiac rehab (31, 32, 33). Severe congestive heart failure may still be a contraindication to aquatic exercise, however (34).
Because the ultimate purpose of the heart as an organ is to pump blood, its ultimate measure of performance is the amount of blood pumped per unit time. Cardiac output is the product of stroke volume times pulse rate per unit time. Submersion to the neck increases cardiac output by more than 30% (15). Output increases by about 1,500 mL/minute, of which 50% is directed to increased muscle blood flow (15). Normal resting cardiac output is approximately 5 L/minute. Maximum output in a conditioned athlete is about 40 L/minute, which is equivalent to 205 mL/beat times 195 beats/minute. Maximum output at exercise for a sedentary individual on land is approximately 20 L/minute, equivalent to 105 mL/beat times 195 beats/minute (24). Because immersion to the neck produces a cardiac stroke volume of about 100 mL/beat, a resting pulse of 86 beats/minute produces a cardiac output of 8.6 L/minute and is already producing increased cardiac work. The increase in cardiac output appears to be somewhat age dependent, with younger subjects demonstrating greater increases (59%) than older subjects (22%) (35). The increase is also highly temperature dependent, varying directly with temperature increase from 30% at 33°C to 121% at 39°C (17). Recent research has shown that conditioned athletes demonstrate an even greater increase in cardiac output than untrained control subjects during immersed exercise and that this increase was sustained for longer periods than in the control group (36). Therefore, the myth that water exercise is not aerobically efficient is faulty; it may be an ideal cardiovascular conditioning medium. There is an emerging body of research on water exercise-induced cardiac output, but significant work needs to be done to delineate the effects of age, gender, temperature, and conditioning, as well as to explain the significant individual response variations. The cascade of cardiovascular responses to immersion is shown in Figure 62-1.
During immersion to the neck, systemic vascular resistance decreases by 30% (15). Diminished sympathetic vasoconstriction produces this decrease, with peripheral venous tone decreasing by 30% from 17 to 12 mm Hg at thermoneutral temperatures (37). Total peripheral resistance decreases during the first hour of immersion and remains low for several hours thereafter. This decrease is related to temperature, with higher temperatures producing greater reductions. This resistance drop decreases end-diastolic pressures. Immersed systolic pressures increase with increasing workload, as they do on land, but these increases appear to be reduced in magnitude when compared with equivalent land-based work (38). Venous pressures also decrease during immersion because less vascular tone is required to support the system.
The effect of immersion on blood pressure has been quite extensively studied. A consistent finding has been the striking individual variation, but trends have emerged that are useful. Very short-term immersion (10 minutes) in thermoneutral temperatures has been found to slightly increase both systolic and diastolic pressures, perhaps as part of the thermal accommodation process (38). By contrast, a pilot study in our institution found that mean arterial pressures demonstrated a 15% to 25% decrease at 5 minutes of immersion. Other studies conducted in carefully controlled environments have found no
effects or actual decreases in pressures during longer immersion periods more typical of therapeutic sessions (39). In an important study for aquatic rehabilitation, Coruzzi et al. (40) found that longer immersion produced significant decreases in mean arterial pressure, with sodium-sensitive hypertensive patients showing even greater decreases (18 to 20 mm Hg) than normotensive patients, and sodium-insensitive patients showing smaller decreases (5 to 14 mm Hg). No studies have demonstrated consistent sustained increases in systolic pressure with prolonged immersion, although several have found no significant decrease. Based on a substantial body of research, the therapeutic pool appears to be both a safe and potentially therapeutic environment for both normotensive and hypertensive patients, with both sodium-sensitive and sodium-insensitive hypertensive individuals demonstrating decreases in pressure during therapeutically customary periods of immersion (41).
effects or actual decreases in pressures during longer immersion periods more typical of therapeutic sessions (39). In an important study for aquatic rehabilitation, Coruzzi et al. (40) found that longer immersion produced significant decreases in mean arterial pressure, with sodium-sensitive hypertensive patients showing even greater decreases (18 to 20 mm Hg) than normotensive patients, and sodium-insensitive patients showing smaller decreases (5 to 14 mm Hg). No studies have demonstrated consistent sustained increases in systolic pressure with prolonged immersion, although several have found no significant decrease. Based on a substantial body of research, the therapeutic pool appears to be both a safe and potentially therapeutic environment for both normotensive and hypertensive patients, with both sodium-sensitive and sodium-insensitive hypertensive individuals demonstrating decreases in pressure during therapeutically customary periods of immersion (41).
In 1989, Gleim and Nicholas (38) found that oxygen consumption (VO2) was three times greater at a given running speed (53 m/minute) in water than on land. Therefore, during water walking and running, only one-half to one-third the speed was required to achieve the same metabolic intensity as on land (38). It is important to note that the relationship of heart rate to VO2 parallels the relationship during land-based exercise, even with accounting for the heart rate decrease in water. Consequently, metabolic intensity in water may be predicted as on land from monitoring heart rate.
Pulmonary System Effects
The pulmonary system is profoundly affected by immersion of the body to the level of the thorax. Part of the effect is due to the shift of blood into the chest cavity, and part is due to compression of the chest wall itself by water. The combined effect is to alter pulmonary function, increase the work of breathing, and change respiratory dynamics.
Functional residual capacity reduces to about 54% of the normal value with immersion to the xiphoid (42). Most of this loss is due to reduction in expiratory reserve volume (ERV), which decreases by 75% at this level of immersion (20). The change in this volume may be readily experienced at poolside: while sitting on the edge of the pool exhale normally, and then expel the rest of the reserve volume forcibly. Take a normal breath and then enter the water to neck level and perform the same
experiment. The difference is very perceptible. Little air remains to exhale at the end point of relaxed exhalation. ERV is reduced to 11% of vital capacity, equal to breathing at a negative pressure of -20.5 cm H2O (42,43). There is some loss of residual volume, which decreases by 15% (42). Vital capacity decreases by about 6% to 9% when comparing neck submersion to controls submerged to the xiphoid (20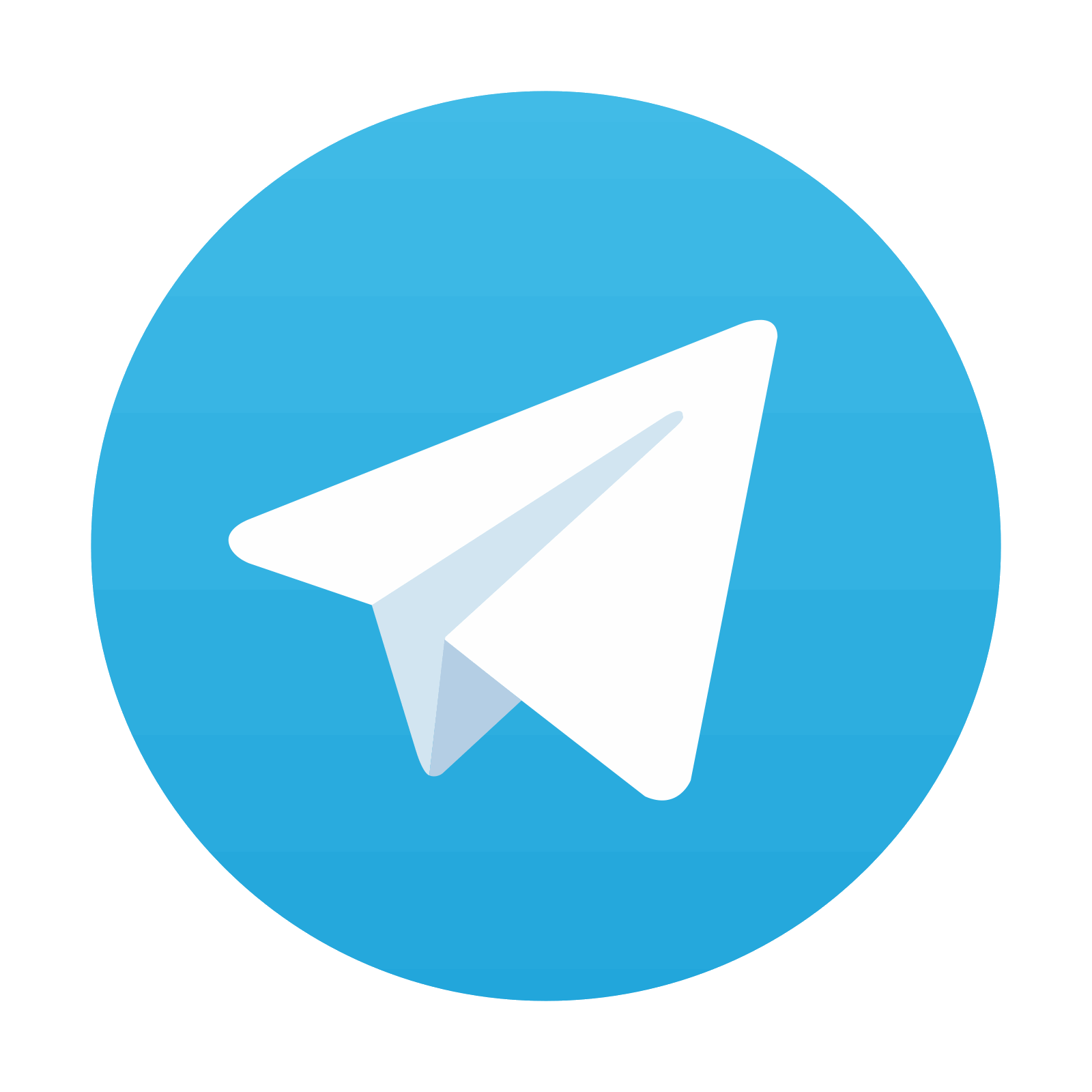
experiment. The difference is very perceptible. Little air remains to exhale at the end point of relaxed exhalation. ERV is reduced to 11% of vital capacity, equal to breathing at a negative pressure of -20.5 cm H2O (42,43). There is some loss of residual volume, which decreases by 15% (42). Vital capacity decreases by about 6% to 9% when comparing neck submersion to controls submerged to the xiphoid (20
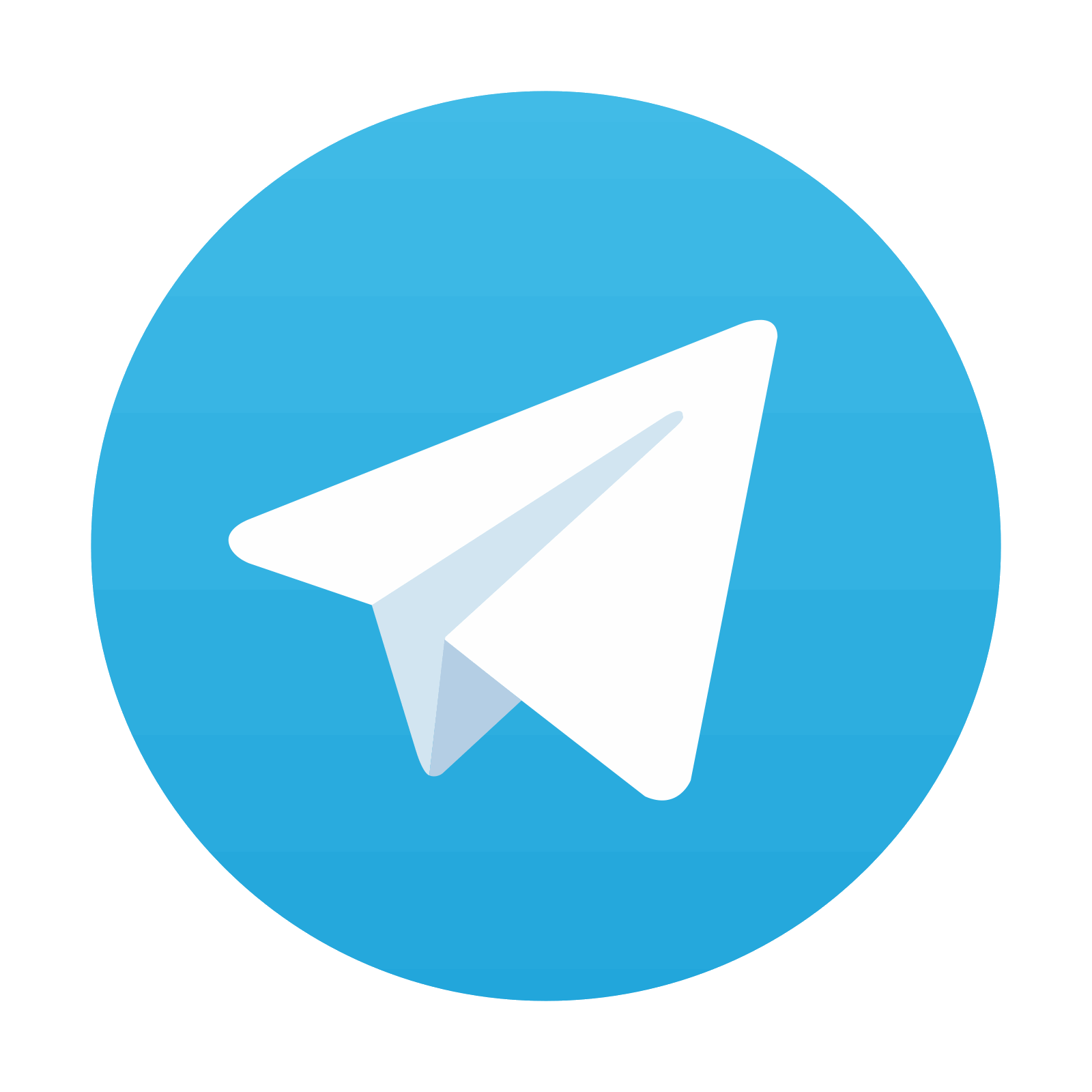
Stay updated, free articles. Join our Telegram channel
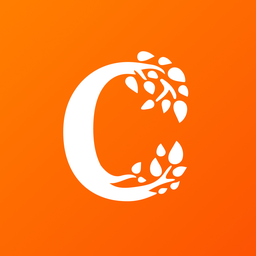
Full access? Get Clinical Tree
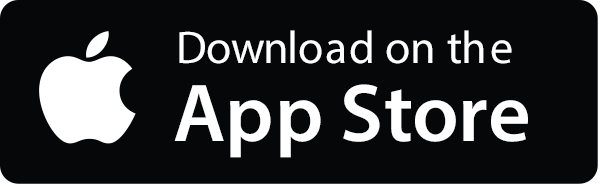
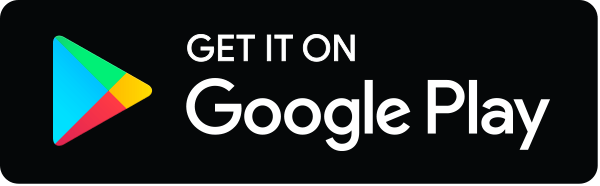
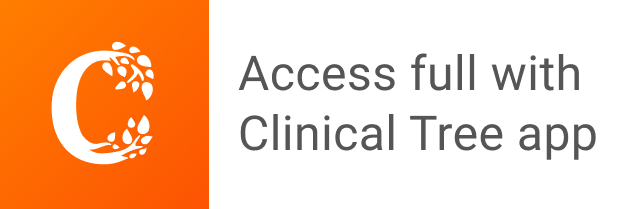