31 Key Points 1. SCI researchers utilize a wide range of animal models that incorporate various aspects of injury progression and inform on pathophysiological progression, mechanisms, and potential treatments for SCI. 2. Different animal models have different strengths and weaknesses; understanding these characteristics, along with any underlying assumptions, is essential in drawing the proper conclusions from a given model. 3. Animal models of SCI can focus on recapitulating clinical correlates or testing proof-of-concept variables that are applicable to SCI, and each has its importance to advancing the field. 4. SCI researchers have a variety of tools to assess animal models of SCI, including histology, motor and sensory behavioral tasks, and electrophysiology. The goal of all spinal cord injury (SCI) models is to create a reproducible, standardized, and consistent method of assessing damage and recovery of the spinal cord. Animal models of SCI are an important tool for understanding the pathophysiological progression, mechanisms, and potential therapeutic intervention points for SCI. This chapter reviews the principal animal models of SCI and their clinical correlates. Additionally, the text addresses proof-of-concept models for remyelination, ischemia, and regeneration, as well as histological and behavioral assessment issues in these models (Table 31.1). A variety of species have been utilized in SCI research (Table 31.2). The majority of work in recent years has been done in rats, mainly due to the low cost and established methods of functional behavioral analysis; however, the mouse has seen increased use, primarily due to the advantage of genetic manipulations and the development of new injury devices. Although these species fulfill an extremely useful role for research purposes, larger-scale animal models may have a role in clinical translation for some therapies,1 particularly in the context of scaling from mouse, to rat, to human spinal cord (Fig. 31.1). Nonhuman primate models of SCI are rare in comparison to rodent models, mainly due to the high cost, specialized care required, and difficulty of producing reproducible lesions.2 In parallel, smaller animal species have been critical to advancing the identification of new molecules and pathways in central nervous system (CNS) regeneration. Table 31.2 Summary of Animals Used in Spinal Cord Injury Research Animal Features Limitations Rodents Larger N possible (lower cost and technical hurdles) Reproducible injuries Well-defined behavioral assessments Clinically similar respiratory neuroanatomy Clinically similar cellular immune response Small size and shorter lifespan Strain differences add complexity Some differences in vasculature and tract locations and features from humans Some differences in humoral immune response from humans Lack paw and digit dexterity Quadrupedal locomotion: different musculoskeletal anatomy; strong role for forelimbs in locomotion Mouse High potential for gene manipulation Immunodeficient models for assessment of human cell therapies in absence of rejection confounds Smallest size Lesion epicenter forms fibronectin-filled scar, unlike many clinical cases Rat Best-characterized injury and behavioral assessments Some immunodeficient models Lesion epicenter cavitation Reduced potential for gene manipulation Larger mammals Larger size provides scaling information for regeneration, transplanted cell migration and procedural safety Similar vasculature and tract organization to humans Hindlimbs drive locomotion Smaller N possible (higher cost and technical hurdles) Lack of standardized injury models and established behavioral assessments Very limited potential for gene manipulation Cat Historically important model Some standardization of injury and behavioral assessment Dog Potential for assessment of naturally injured domestic dogs via veterinary clinics Mini pig Closely mimics human vasculature Nonhuman primates Larger size provides scaling information for regeneration, transplanted cell migration, and procedural safety Highly similar vasculature and tract locations and features to humans Genetic similarity to humans (good for pharmacological interventions) Bipedal locomotion assessments Physical therapy interventions Assessment of hand dexterity Complex cortical circuits for simple motor tasks mimic human plasticity potential Motivational aspects can be evaluated Very low N possible (highest cost, technically difficult, not available to all researchers) Increased ethical considerations Difficult, although sensitive, behavioral measures Very limited potential for genetic manipulation High hurdle to achieve adequate long-term immunosuppression for xenograft transplantation studies Marmoset Established contusion model Small primate (rodent equivalent) Macaque Large primate Injury limited to partial transection, while contusion is most common clinically Fig. 31.1 Size comparison of mouse, rat, and human spinal cords. Note the much larger human spinal cord and the possible challenges that this difference may create for translation from rodents to humans, especially with regard to cell transplantation, lesion dynamics, and distance traversed by regenerating fibers. The critical criterion required of an animal model is how well it addresses or can be applied to human SCI. Injuries in humans are a heterogeneous mix of types and severities at different levels, accompanied by differing degrees of functional deficits. Consequently, animal models need to be capable of incorporating these factors. The first factor a model must address is the injury type and severity. Injury types in humans have been classified into four broad categories: solid cord injury (10% of cases), contusion/cavity (49% of cases), laceration (21% of cases), and massive compression (20% of cases), with the majority of these injuries being anatomically incomplete.3 Consequently, animal models have been focused on mimicking these types of injuries and are discussed based on their method of injury here. Additionally, the ability to create graded injuries of varying severities and to detect these differences in a behavioral and histological manner is critical for translatable success to the varied human SCI population and has been a chief criterion for the development of these models. Another important factor to address is injury level. Many animal models have utilized midthoracic injuries, resulting in various degrees of hindlimb paralysis and trunk instability. Since the species used are predominantly quadrupeds, this level of injury has significantly different behavioral outcomes than what can occur in bipeds, like humans. The majority of human injuries are in the cervical region,3 and a recent survey of individuals with SCI has shown that regaining the ability to walk is lower in priority than other functions, such as arm movement, bladder and bowel control, and sexual function.4 Thus it is critical that animal models be designed to address these needs, and there has been a recent surge in efforts to develop cervical injury and assessment models. The biggest obstacle to the implementation of consistently reproducible cervical SCI models has been with regard to gaining access to the spinal cord due to the more complex musculature and neuroanatomy at that level. Regardless, the majority of injury paradigms and models discussed throughout this chapter can be applied at any desired vertebral level, given the proper training and reproducibility. Furthermore, induction of SCI in animal models is performed under anesthesia in the absence of other conflicting variables. In contrast, many human SCI cases are accompanied by comorbidities and secondary trauma (e.g., in the case of automobile accidents, one of the leading causes of SCI).3,5 These situations lead to quite different conditions between clinical reality and animal models. Finally, some consideration should be given to what has been learned about differences in SCI among different species. First, despite a great deal of conservation of neuroanatomy and function across species, there are distinct differences in the transition from rodent to primate to human.6 Furthermore, even rodents have their differences: rats and mice have been shown to have different inflammatory reactions following SCI,7 and different strains of mice have been shown to have different levels of complement activity,8 excitotoxicity, secondary degeneration, and wound healing following SCI.9 Finally, age has been shown to be a critical factor in humans10 as well as rats,11,12 with increased age being correlated to higher mortality and greater locomotor and histological deficits. This is particularly critical because the vast majority of rodent SCI models are conducted in young adult animals, 8 to 12 weeks of age, whereas the average age of human clinical SCI is currently 40.5 Ultimately, it is important to be aware of the strengths and weaknesses of a model and the assumptions being made regarding it as well. Although some models are more clinically translatable, all of the animal models presented here have strongly shaped what is known about SCI and have influenced where treatments are headed in the future. Many commonly used animal models of SCI mimic the injury, pathology, and behavior observed in human patients. This section focuses on contusion, compression, transection, and root avulsion models and their individual contributions to the understanding of the underlying mechanisms and treatment of SCI in humans. Laminectomies are frequently performed as the first step of most animal injury models. Care should be taken to ensure that extensive damage to the musculature or inadvertent damage to the spinal cord is avoided when exposing and removing the vertebrae. Additionally, the musculature and skin need to be sutured properly to avoid the development of kyphosis in animals. Ultimately, with training and practice, laminectomies can be incident-free and a reliable and reproducible way of exposing the spinal cord for further experimentation. Contusion models are widely used in an effort to mimic what occurs in the majority of human injuries.3 These models all utilize some form of blunt trauma, followed by compression of the spinal cord, which results in the development of a progressive lesion that is very similar to that seen in human SCI.13 It should also be noted that the dura is not disrupted in these injuries, and none include stretching or shearing, which is sure to be a clinical factor. The focus presented here is on the blunt injury models that have the highest prevalence within the scientific literature. The clip compression model utilizes a modified aneurysm clip to produce consistent and reproducible injuries. Compression time can be varied to alter injury severity, or clips with different compressive force can be used while keeping time consistent.14 Alternatively, the use of graded forceps has also been described as a way to create a range of injuries in mice that can be visualized both behaviorally and histologically.15 When used properly, either of these models is a cost-effective method of creating reproducible injuries across a variety of species. However, they lack some of the more advanced features and data output of recently developed computer-controlled devices. Computer-assisted contusion devices are advantageous due to their consistency and the amount of data they report, which can be used to determine injury reliability, efficacy, and animal exclusions at the time of surgery. The OSU impactor16 was first used in rats, and was later modified to improve consistency and accommodate the use of mice.17 It utilizes the amount of cord displacement as a variable for injury and reports the total amount of displacement and force transmitted to the spinal cord. The NYU impactor18 is a weight-drop method of inducing contusion injuries in rats. Height is varied to affect injury severity and a variety of parameters are reported, including rod impact velocity, compression rate and distance, and the total force applied to the cord. The Infinite Horizon Impactor (Precision Systems and Instrumentation, Fairfax Station, VA)19 utilizes a computer-controlled probe to vary the amount of user-defined force that is applied to the spinal cord of a variety of species. The device reports a wealth of parameters, including the actual force applied, the velocity at maximum force, and the displacement of the cord, and it also generates a graph that plots changes in force and displacement as a function of time. As noted in the introduction, age, like strain and species variations, is likely an important variable in both the initial injury and recovery from SCI. Contusion injury models have shown greater deficits in functional recovery in aged animals after SCI, but, due to the complex nature of these models, the biological rationale behind this difference is difficult to determine.11,12 Complete transection models involve the severing of the spinal cord, resulting in a complete injury, often called a “spinalized” animal. Clinically, this model mimics a complete laceration of the spinal cord, which can occur from stabbing or bullet wounds, but these injuries are often incomplete and are rare.3 However, the complete nature of the injury provides a clean way for researchers to judge axonal regeneration and behavioral recovery. Care must be taken to ensure that the injury is complete or spared axons can be mistakenly counted as regenerating axons.20 Retrograde and anterograde tracers are utilized to visualize the presence of regenerating versus spared axons. The distinct, localized trauma can also be advantageous for studies looking at less complex injuries and interested in a specific scientific question regarding axonal regeneration, but the model does not show the same extent of pathophysiological changes seen in contusion injuries.21 Finally, it should be noted that behavioral recovery due to intervention after complete transection should be confirmed by retransection to avoid the possibility of native locomotion reported in fully transected animals.22 Partial transections are generally utilized to examine specific tracts or regions of the spinal cord. Clinically, this model is comparable to incomplete lacerations of the human spinal cord, albeit in a much more clean and precise fashion. Although these models carry some of the same limitations as complete transection, they do have some advantages. A partial lateral transection allows for comparison with the uninjured contralateral side in the same animal. Furthermore, specific tracts and pathways can be targeted depending on the scientific question or clinical relevance. Due to the incomplete nature of the injury, it is important to distinguish between spared axons and potential regenerating axons even more so than with a complete transection. Retrograde and anterograde tracers can aid in this determination. One important confound with regard to behavior for these animals is that recovery can sometimes be accomplished through the use of compensation and plasticity of spared regions.23 Another animal model of SCI is root avulsion, in which motoneurons, parasympathetic neurons, and dorsal root ganglia (DRG) cells are axotomized at the interface between CNS and peripheral nervous system (PNS).24 Because of the increased regenerative capacity of the PNS versus the CNS, the location of the injury in this model poses a unique scientific question. Following laminectomy, the injury is performed using forceps to deliver longitudinal traction to either dorsal25 or ventral roots26 of the brachial plexus,27 lumbosacral plexus,28 or cauda equina.26 The clinical correlate of this model is often a complex injury involving rupture and avulsion of both dorsal and ventral roots resulting from motor vehicle accidents or complications during birth. Lumbosacral plexus avulsions are much less common clinically than brachial plexus avulsions, due to the anatomy of the spinal column.29 The animal model shares the clinically described deficits in motor, sensory, autonomic, bladder, and bowel function.30,31 The emergence of pain and the dramatic loss of neurons after avulsion is also evident in these animals, similar to the human condition.29 The root avulsion injury model has been used to characterize the inflammatory response and the number of motoneurons remaining after progressive loss following axotomy.26 Clinically, this SCI animal model was pivotal in developing root reimplantation surgery, which is today the only successful treatment for not only brachial plexus injuries but also any SCI in humans. Reimplantation of the avulsed ventral root (or PNS graft) into the lateral white matter of the spinal cord (or into the ventral root exit zone) has been demonstrated to lead to recovery of motor and bladder function accompanied by pain reduction within 1 year in both animals and humans.29,32 A combinatorial approach including neurotrophin treatment to reduce motoneuron death may prove to be most beneficial clinically.33 In addition to traditional animal models with clinical SCI correlates, several other commonly employed models include chemical demyelination, photochemical lesions, and peripheral conditioning injuries. These proof-of-concept models allow researchers to isolate a process of interest in SCI and to study this process in a system with relatively few variables before moving to a more complex, clinically translatable model. Thus, studies using these paradigms are integral to our understanding of the molecular mechanisms of processes associated with SCI or recovery from injury, including myelination, ischemia, and regeneration. The myelination component of SCI has been studied using ethidium bromide (EB) or lysolecithin (LL) to create focal demyelination lesions in animals in the absence of mechanical SCI.34 These models induce the synchronous and rapid demyelination of axons as a result of oligodendrocyte death, with little direct axonal damage. In addition, there is evidence for depletion of the endogenous oligoprecursor population within the lesion site, and spontaneous, essentially complete, host-mediated remyelination takes place over a period of 2 to 3 weeks in animals lesioned at a young age (8 to 10 weeks). Further, transplantation of a variety of oligodendrocyte precursor cell (OPC) populations results in spinal lesion remyelination.34 A significant variable that has emerged is the role of age at time of lesion, with age-related deficits in remyelination seen in rodents over 5 months in comparison with those under 3 months of age.34,35 As mentioned previously, a reduction in functional recovery and myelination with age has also been demonstrated in the contusion model, demonstrating that proof-of-concept models can yield valuable mechanistic insight to the more complex clinical models. One method of initiating an ischemic lesion is through the use of rose bengal, a photosensitive dye. The dye is injected into animals intravenously and activated by irradiation of the spinal cord with a 560 nm laser beam.36 This ischemic lesion differs from surgical occlusion models in that platelet aggregation is the primary initiator of the ischemic event. Numerous groups have characterized the morphological and electrophysiological aspects of the lesion and have shown that lesion size, severity, and location can be controlled.37 Further-more, the surgery requires no laminectomy, due to the translucent properties of rodent bone. Finally, the injury model lacks the complications of hemorrhaging seen in mechanical trauma models. However, the model does not mimic the type of injury commonly seen in human patients, the contusion injury. Nonetheless, it is a useful tool for focusing on the ischemic component of SCI. Peripheral and central branches of sensory neurons are known to respond differently to injury, with marked regeneration of the peripheral axons and no regeneration of the central axons contained within the dorsal column of the spinal cord.38,39 This lack of central regeneration is due to a reduced intrinsic growth state of CNS neurons combined with inhibitory cues from CNS myelin and the glial scar. CNS axons have been demonstrated to grow into a PNS tissue graft after injury in rodents and humans, which points to the importance of the microenvironment in nervous system regeneration.29,38,40 However, conditioning peripheral nerve lesions in rats and mice have recently been demonstrated to increase axonal regeneration through the injured tissue and rostral to the site of dorsal column transection without the aid of a PNS graft.41,42 This central regeneration was originally demonstrated with sciatic nerve transection 1 week prior to dorsal column injury42 (“prepriming”), and more recently with sciatic nerve transection at the time of dorsal column injury and again 1 week after dorsal column injury41 (“priming plus postpriming”). Although the latter paradigm is more clinically relevant with regard to timing, a peripheral nerve lesion cannot be conducted on human patients for ethical reasons. In addition, motor regeneration and functional motor recovery would not be expected to result from sensory regrowth. Therefore, the usefulness of this animal model lies in the elucidation of the mechanism of a rare instance of CNS regeneration, and the use of that knowledge to augment regeneration after diverse spinal cord insults. Considering the difference in PNS versus CNS regenerative capacity, and the elongation of central DRG axons after axotomy to both branches (but not to the central axon alone), the mechanism of central regeneration in this model likely involves changes in protein expression at the level of the DRG cell soma. Indeed, many signaling proteins and transcription factors appear to be involved in regeneration of sensory afferents in the spinal cord.43–45 Interestingly, the conditioning lesion effect on central axon regeneration is mimicked by intraganglionic injection of a cyclic adenosine monophosphate (cAMP) analogue.46 It is important to note that, although central sensory axons from conditioned DRGs regenerate through a spinal cord lesion, many axons also adopt tortuous trajectories within the injured tissue and do not emerge rostrally.42 Also, the functional capacity of the regenerated sensory afferents is questionable because they appear to remain in a chronic pathological state according to electrophysiological measures, such as conduction velocity.47 Nevertheless, the DRG conditioning lesion is a powerful model of CNS regeneration, allowing for the exploration of regenerative mechanisms and of the impact of environmental and intrinsic factors on spinal cord regeneration, possibly leading to future SCI treatments. A parallel and widely used model of CNS regeneration is the optic nerve crush model, in which lens puncture combined with forcep crush of the optic nerve induces axonal elongation into the optic nerve.48 This model has the advantages of being completely contained within the CNS, and the relative simplicity of the optic nerve compared with the spinal cord. Many of the same proteins and processes, including cAMP, are involved in both models of regeneration.49 The assessment of recovery after SCI may involve histology, motor and sensory behavioral tasks, and occasionally electrophysiology. Common histological considerations include lesion volume and tissue sparing, but neuroanatomical tracing of regenerating fibers and quantification of inflammatory cells are also used, depending on the model and hypothesis being addressed. It is important to note that variations in total spinal cord volume can accompany injury, and the recovery from injury.11 Importantly, unbiased stereological quantification is equipped to account for these changes in volume, and therefore provides a more accurate evaluation of histopathological parameters. Many behavioral tasks are available for the evaluation of deficit and recovery after SCI.50 Behavioral measures should be chosen on the basis of sensitivity to injury level, type, and severity. Therefore, appropriate tasks are chosen based on the outcome variable of interest, and constrained by the methods of the study. A particularly useful tool for screening of hindlimb function in rats is the Basso, Beattie, Bresnahan scale (BBB), in which gross assessment of locomotor recovery is evaluated in an open field, resulting in a score of 0 to 21, with 21 as normal and 0 as completely paralyzed.51 An open-field rating scale has also been developed for mice—the Basso Mouse Scale (BMS).52 Although these open-field measures are an important first step, both suffer from nonlinearity and differences in sensitivity across the broad spectrum of recovery. Accordingly, linearly valid secondary measures, such as gait analysis (e.g., CatWalk, Noldus, Wageningen, The Netherlands),53 kinematic analysis,54 horizontal ladder beam,55 inclined plane,56 grid walking,57 ground reaction force measurement,58 and swim testing,59 can be used to tease apart more subtle differences in recovery. In addition, common behavioral assessments of forelimb function after cervical SCIs include limb hanging,60 grip strength,61 rope climbing,62 paw preference,63 and pellet reaching64 tasks. In addition, Von Frey’s testing65 evaluates mechanical allodynia, whereas Hargreaves, or hot plate, testing66 evaluates temperature sensitivity. Improvement in bladder/bowel and sexual function is of paramount importance to the SCI patient population,4 and hurdles to clinical translation may not be as high for autonomic function as for locomotion, but these outcome measures are widely underassessed in SCI models. Methods for evaluation of micturition,67 erection,68 and autonomic dysreflexia69 do exist and should therefore be assessed in all SCI animal models. Studies employing animal models of SCI are essential for driving the understanding of the underlying mechanisms of the injured or diseased CNS and human clinical functional and pathological deficits. Much of what is known about the human condition is derived from animals, because more stringent controls as well as more potential treatment approaches and exploratory experiments are available to the animal researcher than to the clinician. With little or no scientifically sound treatment options currently available for the majority of SCIs in humans, translation from animal studies, including current and planned clinical trials,70 will prove to be integral in alleviating the functional deficits and pain suffered by spinal cord injured patients in the future.
Animal Models of Spinal Cord Injury
Animal Spinal Cord Injury Models with Clinical Correlates
Laminectomies
Compression and Contusion Models of Spinal Cord Injury
Complete and Partial Transection Models of Spinal Cord Injury
Root Avulsion Models of Spinal Cord Injury
Proof of Concept for Myelination, Ischemia, and Regeneration in Spinal Cord Injury
Chemical Demyelination Models of Spinal Cord Injury
Photochemical Lesion Model of Spinal Cord Injury
Peripheral Conditioning Lesion Model of Spinal Cord Regeneration
Assessments for Animal Models of Spinal Cord Injury
Histology
Behavior
Conclusion
Human SCI is heterogeneous, and animal models allow researchers to control injury-associated variables.
Animal models provide a means for acquiring graded, reproducible injuries from which pathological and behavioral comparisons between treatment groups can be made.
Proof-of-concept animal models of SCI allow for isolation of specific aspects of the clinical picture for treatment proof-of-concept and mechanism questions.
Some animal models of SCI mimic most aspects of the clinical picture, allowing for the evaluation of potential human therapies in a complex environment.
The overall number of human SCI patients and the ability of researchers to acquire histological and electrophysiological data from these patients are very limited, emphasizing the need for animal models.
Stay updated, free articles. Join our Telegram channel
Full access? Get Clinical Tree
Animal Models of Spinal Cord Injury
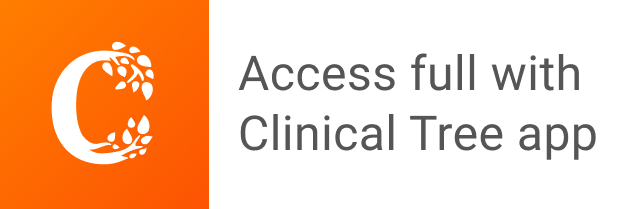