Therapeutic Electrical Stimulation in Neurorehabilitation
Lynne R. Sheffler
Jayme S. Knutson
John Chae
INTRODUCTION
The clinical application of electrical stimulation (ES) to treat neurologic disease may be broadly classified as therapeutic or functional. The purpose of therapeutic ES is to improve the health or voluntary function by inducing physiological changes that remain after the stimulation is used. Therapeutic application of ES may either decrease impairment or prevent further impairment associated with immobility or disuse of a limb or organ system. The use of ES for functional purposes is distinct from therapeutic ES in that the benefits persist only during the period of intervention. The term functional electrical stimulation (FES) refers to application of electrical current to activate paralyzed muscles in precise sequence and intensity as to supplement or replace lost function. In FES applications, stimulation must be “on” to achieve a desired function; therefore, FES systems are usually designed to be worn by the user and are called neuroprostheses because they substitute for lost function. As an intervention in neurorehabilitation, specific applications of FES may have both neuroprosthetic and therapeutic benefit. This chapter is limited to the therapeutic applications of ES to treat the neurologically based disorders most commonly encountered in the clinical practice of Physical Medicine and Rehabilitation. Neuroprosthetic or FES applications of ES is reviewed in the chapter entitled Functional Neuromuscular Electrical Stimulation.
HISTORY
The history of therapeutic ES dates back to 3,000 BC; archeological evidence suggests that Egyptians knew of the powerful electrical shock emitted by an electric catfish found in the Nile River. In ancient Egyptian tombs, hieroglyphics have been discovered which depict the Malapterurus Electricus (1), a species of catfish now known to possess electric organs innervated by a pair of electromotoneurons located within the cervical spinal cord. In 43 AD, Scribonius Largus, a physician to the Roman emperor Claudius, provided the first written documentation of the therapeutic application of ES (2). In the book entitled, “Compositions Medicamentorum,” he detailed the use of the electric torpedo fish for the transient relief of pain associated with headaches and gout. The torpedo fish, which could produce electric shocks between 100 and 150 V, was applied either directly on the patient or placed in a pool of water in which the patient stood to provide therapeutic benefit.
It was not until the middle of the 18th century that potential medical applications of electricity became a focus of study. The Leyden jar, invented in 1745 by Pieter van Musschenbroek (1700 to 1748), was an early device for the storage of electricity which helped early researchers to try various therapeutic applications of electricity. In the 1750s, Benjamin Franklin noted the amnestic effect of electricity applied to the brain (3) and experimented with ES in an attempt to restore motor movement in stroke survivors (4). Luigi Galvani’s description of his “animal electricity” experiments in 1786 and the invention of the voltaic cell by Allessandro Volta (1745 to 1827) significantly contributed to the foundation for modern electrophysiology. Michael Faraday (1791 to 1867) in 1831 performed a series of experiments, establishing the concept of electromagnetic induction. His characterization of the relationship between a DC electric current and a magnetic field further laid a foundation for the therapeutic use of electricity. Duchenne De Boulogne (5) (1806 to 1875) in the mid-1800s used ES both as a therapeutic agent as well as a diagnostic agent to map functional anatomy. Despite these advances, medical skepticism of the therapeutic value of ES persisted through the end of the 19th century.
The advent of the 20th century heralded significant advances in the therapeutic applications of ES. ES of the human sensory cortex was first reported by famed neurosurgeon Harvey Cushing (1869 to 1939) in 1909 (6). Walter Rudolph Hess (1881 to 1973) reported “affective responses” to hypothalamic stimulation in 1928 (7). In 1969, D.V. Reynolds described the analgesic effect of ES on the periaqueductal gray matter of the brain in an animal model (8). Today, transcutaneous electrical nerve stimulation (TENS) and neuromuscular electrical stimulation (NMES) systems are commercially available and routinely employed for the treatment of neuropathic pain and paralysis. Spinal cord stimulation (SCS) and deep brain stimulation (DBS) have been shown to be safe and efficacious for the treatment of various neurological symptoms including neuropathic pain and movement disorders. The burgeoning field of Neuromodulation encompasses the reversible therapeutic modulation of central, peripheral, and autonomic nervous system activity via ES and proposes to provide
practical, clinical treatment options for neurologically disabled patients in the 21st century.
practical, clinical treatment options for neurologically disabled patients in the 21st century.
TENS FOR PAIN
TENS is a widely used form of electroanalgesia based on the “gate control theory of pain” postulated by Melzack and Wall in 1965 (9). The “gate theory” of pain control states that stimulation of large somatosensory fibers in peripheral nerves or at the dorsal column can effectively inhibit the central transmission of nociceptive signals carried by small, unmyelinated C-fibers from the periphery. Activation of low-threshold myelinated fibers essentially “closes the gate” at the presynaptic level of the dorsal horn of the spinal cord to produce transient pain relief. In 1967, Wall and Sweet (10) demonstrated the electroanalgesic effect of transcutaneous sensory stimulation applied at 100 Hz to patients with chronic cutaneous pain.
The proposed mechanism of neuromodulation via TENS remains controversial. The literature supports a primary effect via presynaptic inhibition at the level of the dorsal horn of the spinal cord as evidenced by changes in neurotransmitter levels associated with application of TENS. In an animal model, high-frequency TENS has been shown to activate delta-opioid receptors and thus reduce the release of glutamate and aspartate at the level of the spinal cord which would otherwise be elevated in association with joint inflammation (11). TENS has also been shown to reduce production of substance P in the dorsal root ganglion and thus suppress nociception via C-fibers in the peripheral nerve (12). Central mechanisms have been proposed to explain the effect of TENS including a neuromodulating effect via descending supraspinal sites such as the rostral ventromedial medulla (13). In a study examining the effect of sensory input on corticospinal excitability, short-term high-frequency TENS was found to decrease human motor cortex excitability (14). Lastly, research has suggested that TENS-mediated hypoalgesia may, in part, be the result of a direct peripheral effect (15).
The first modern TENS unit (16) received a U.S. patent in 1974. A TENS unit is a battery-powered electronic pulse generator that provides low-voltage electrical current through transcutaneous electrodes. A constant voltage stimulator allows for fluctuations in current intensities that may occur with variable impedance of the electrodes, skin, and tissues. A TENS unit is programmable and optimal settings are determined based on the symptomatic response of the individual patient. The parameters for prescription of TENS include stimulation intensity (mA), pulse frequency (range 2 to 250 Hz), and pulse duration (50 to 1,000 µs). Conventional TENS is the most commonly applied mode of stimulation for rapid-onset, localized anesthesia. Conventional TENS is characterized by high stimulation frequency (>100 Hz), low intensity (10 to 30 mA), and short pulse duration (50 to 100 µs). Acupuncture-TENS is characterized by high intensity stimulation (sufficient to evoke muscle contraction) delivered at low frequency (<4 Hz) and longer pulse duration (>200µs). While acupuncture-TENS may produce a more sustained analgesia, tolerance is less than with conventional TENS. Lastly, pulsed (burst) TENS is essentially a combination of conventional and acupuncture-TENS, and is characterized by bursts of high frequency stimulation (>100 Hz) delivered in bursts at lower frequency (<4 Hz).
No specific advantage is established for pulsed TENS as compared to conventional TENS. The clinician determines the placement of transcutaneous electrodes based on the pain location and may be influenced by trigger points, cutaneous nerve paths, and location of traditional acupuncture sites. For low back pain, for example, transcutaneous electrodes may be placed several centimeters apart either on or adjacent to the painful region, with stimulation being delivered with a pulse frequency of 80 Hz, pulse duration of 100 µs, and amplitude set to the submotor threshold, which varies widely from patient to patient. The onset of analgesia is immediate and may persist beyond the interval of time that the TENS unit is stimulating.
Indications for the use of TENS include both neurogenic pain (deafferentation pain, phantom pain, sympathetically mediated pain, postherpetic neuralgia, trigeminal neuralgia, atypical facial pain, brachial plexus avulsion) and musculoskeletal pain (joint pain associated with rheumatoid arthritis, osteoarthritis, acute posttraumatic pain). Placement of TENS electrodes is contraindicated on or near the eyes, in the mouth, transcerebrally, over the carotid sinuses (vasovagal reflex), anterior neck (laryngospasm), on areas of decreased or absent sensation, or over the trigeminal nerve (if history of herpes zoster induced trigeminal neuralgia). TENS should not be used in epilepsy or pregnancy, or in patients with an implanted cardiac pacemaker or defribrillator due to risk of interference or failure. Skin irritation and electrical burn are the most commonly reported complications, but in general, TENS is well-tolerated.
Despite widespread usage and a 40-year history as an adjunctive treatment to pharmacologic pain management, the therapeutic efficacy of TENS remains controversial. A recent Cochrane review (17) analyzed 25 randomized clinical trials (RCTs) (128 subjects) to determine the efficacy of TENS for chronic pain. Trials were included that compared high-frequency TENS with (a) sham TENS (control), (b) no treatment (control), and (c) low-frequency TENS. In 13 of 22 controlled studies, there was a positive analgesic outcome in favor of TENS. No difference in analgesic efficacy was found in seven of nine studies which compared high- and low-frequency TENS. A second Cochrane review (18) analyzed four RCTs (n = 585 subjects) to determine the efficacy of TENS as compared to placebo for the specific treatment of chronic low back pain. A qualitative synthesis was completed as clinical heterogeneity prevented the use of meta-analysis. The authors noted that there was conflicting evidence about whether TENS was beneficial in reducing back pain intensity and back-specific functional status. They concluded that the evidence from the small number of placebo-controlled trials did not support the use of TENS in the routine management of chronic LBP. In summary, TENS is a noninvasive, widely used modality for
pain that is easy to apply with relatively few contraindications. However, high quality, randomized, controlled clinical trials remain necessary to definitively assess the therapeutic role of TENS in the management of pain.
pain that is easy to apply with relatively few contraindications. However, high quality, randomized, controlled clinical trials remain necessary to definitively assess the therapeutic role of TENS in the management of pain.
NMES FOR MOTOR RELEARNING
Motor relearning is defined as the reacquisition of motor skills following central nervous system injury. Research on central motor neuroplasticity supports a primary role of goal-oriented, active repetitive movement training to enhance motor relearning. Asanuma and Keller demonstrated that ES of the somatosensory cortex alone or in conjunction with thalamic stimulation in an animal model induces long-term potentiations (LTP) in the motor cortex (19). They hypothesized that proprioceptive and cutaneous afferent impulses associated with repetitive movements induce LTP in the motor cortex, which then modify the excitability of specific motor neurons and facilitate motor relearning (20). Consistent with this hypothesis, nonhuman primate research has demonstrated that after local damage to the motor cortex, goal-oriented, active repetitive movement training of the paretic limb shapes subsequent functional reorganization in the adjacent intact cortex, and that the undamaged motor cortex plays an important role in motor relearning (21). Repetitive movements that require the development and practice of new motor skills are the kinds of behavioral experiences that induce long-term plasticity in motor maps (21). When animals are trained to perform new tasks such as retrieving food pellets from a small well (22, 23, 24) or a rotating well (25), there is evidence of task-specific cortical reorganization. However, repetitive movement tasks that do not require skill acquisition (i.e., motor tasks that are already mastered and, therefore, are easy to carry out and require minimal or no cognitive effort) are not associated with any significant changes in the motor cortex (24,25).
The use of NMES-mediated goal-oriented repetitive movement therapy for motor relearning is predicated on repetitive movement therapy research. Acute administration of ES to a peripheral nerve activates both sensory and motor structures in the brain (26,27) and reduces the intracortical inhibition (28,29). Functional MRI (fMRI) studies show cortical responses to NMES-facilitated movement in both the upper (30) and lower (31) extremities, which suggests that repetitive movement therapy mediated by NMES has the potential to facilitate motor relearning via cortical mechanisms.
NMES may also facilitate motor relearning via spinal mechanisms. Rushton theorized that the corticospinal-anterior horn cell synapse is a Hebb-type, modifiable synapse and that it can be modified by NMES (32). Originally proposed in 1949, “Hebb’s rule” (33) states: “When an axon of cell A… excite(s) cell B and repeatedly or persistently takes part in firing it, some growth process or metabolic change takes place in one or both cells so that A’s efficiency as one of the cells firing B is increased.” The synapse is thought to be strengthened by the coincidence of presynaptic and postsynaptic activities. Under normal circumstances, neural activity in the pyramidal tract easily discharges the anterior horn cells and the strength of the presumed Hebb-type pyramidal tract/anterior horn cell synapse is maintained by this traffic. However, following brain injury, neural activity in the pyramidal tract is significantly reduced. Failure to restore this traffic leads to “decorrelation” of presynaptic and postsynaptic activities, which weakens the synapse. Rushton suggested that NMES-mediated antidromic impulses provide an artificial means to synchronize presynaptic and postsynaptic activity in the affected population of anterior horn cells. Accordingly, he predicted that combining NMES with simultaneous voluntary effort is an effective means of facilitating motor relearning.
Regardless of cortical or spinal mechanisms, experimental and theoretical considerations suggest that the necessary prerequisites for NMES-mediated motor relearning include repetition, novelty of activity, concurrent volitional effort, and high functional content.
Types of NMES for Motor Relearning
Three NMES paradigms have been used for motor relearning: cyclic NMES, EMG-mediated NMES, and as a neuroprosthesis. Cyclic NMES provides repetitive activation of muscles without input from the stroke survivors. The activity is novel in that the stroke survivor has difficulty performing the task without the NMES; however, the task is not functionally relevant. EMG-mediated NMES couples cognitive intent and NMES-mediated muscle contraction. This approach may be applied to patients who can partially activate a paretic muscle but are unable to generate sufficient muscle contraction for adequate exercise or functional purposes. The approach incorporates novel tasks and includes cognitive investment, but the task is not functionally relevant. Neuroprostheses provide repetitive movements in the context of functional tasks that are novel; thus, neuroprostheses have a theoretical advantage over both cyclic and EMG-mediated NMES for motor relearning.
Upper Limb Applications
RCTs have investigated the efficacy of cyclic NMES in enhancing upper-limb motor relearning (34, 35, 36, 37, 38). Transcutaneous electrodes are typically placed over the motor points of the finger and wrist extensors. Four studies reported improved outcomes in motor impairment at the end of treatment, with mild to moderately impaired subjects benefiting most. Two acute stroke studies reported sustained effect (36,37) and two studies reported improvements of activity limitation at the end of treatment (37,38).
EMG biofeedback, position, or EMG-triggered NMES have been evaluated for upper limb motor relearning in six controlled trials (39, 40, 41, 42, 43, 44). All six studies demonstrated improved outcomes in motor impairment at the end of treatment; one study showed a sustained effect at 9 months posttreatment (40). Two studies demonstrated decreased activity limitation (41,44). Finally, three studies reported evidence of central mechanisms using neurophysiologic assays including reaction time and fMRI (42, 43, 44).
The initial review of these studies suggested that NMES is efficacious in reducing motor impairment but not activities limitation (45). The authors suggested that the effect is more significant for those with milder impairments. A subsequent review by the same group concluded that EMG-mediated NMES may be more effective than cyclic NMES (46).
However, a more recent meta-analysis concluded that EMG-mediated NMES was no more efficacious than “usual care” in facilitating motor relearning (47). They noted that most studies were with chronic stroke survivors and results might be different among acute stroke survivors. Consistent with this conclusion, two small RCTs failed to demonstrate the superiority of EMG-mediated NMES over cyclic NMES (48), or usual care (49) among chronic stroke survivors. While acute studies are ongoing, at present it remains uncertain whether cyclic and EMG-mediated NMES are efficacious in facilitating upper limb motor relearning among chronic stroke survivors.
There is emerging evidence that hand neuroprostheses may facilitate motor relearning in the poststroke patient population. Two recent RCTs using a hybrid brace-NMES device that incorporates surface electrodes into a brace for hand grasp and release showed significant improvements in motor impairment (50,51). A third RCT of multichannel NMES also resulted in significant improvement in upper limb motor function (52). Several novel neuroprosthesis approaches with encouraging preliminary results are presently under investigation, including injectable microstimulators (53), contralaterally controlled surface FES (Fig. 71-1) (54), and the incorporation of work stations (55). Additional studies are needed to confirm the motor relearning effect of hand neuroprostheses, especially with respect to translation to functional abilities.
Lower Limb Applications
Studies of stroke patients treated with cyclic lower limb NMES have demonstrated enhanced walking ability, increased maximal isometric contraction of the ankle dorsiflexors and plantarflexors, increased dorsiflexion torque, increased agonist EMG activity, and decreased EMG co-contraction ratios (56, 57, 58). In one controlled RCT, acute stroke survivors treated with lower extremity NMES and on standard rehabilitation for 3 weeks were more likely to return to a home setting at discharge from rehabilitation compared to controls who did not receive the NMES (58).
The potential motor relearning effect of lower limb neuroprostheses has been evaluated. After using a single channel peroneal nerve stimulator (PNS), Lieberson et al. commented, “On several occasions we observed, after training with the electrophysiologic brace…patients acquire the ability of dorsiflexing the foot by themselves” (59). Since then, case studies using either implanted or transcutaneous electrodes have described similar post-FES observations of improved ambulatory function, more normal EMG muscle activation patterns, emergence of EMG signals in previously silent muscles, increased strength of EMG activity, and decreased co-contraction of antagonist muscles (60, 61, 62, 63, 64, 65, 66). A recent meta-analysis of the effect of nonimplanted FES devices on gait speed concluded that FES was effective in improving poststroke gait speed (67). However, studies with implantable PNSs have yielded conflicting results (68,69).
Since gait deviation is not limited to ankle dysfunction, multichannel transcutaneous stimulation systems have been investigated in hemiplegia (70, 71, 72). However, the therapeutic application of transcutaneous systems becomes more difficult due to limited muscle selectivity, poor reliability of stimulation, and pain of sensory stimulation. Therefore, percutaneous stimulation systems, which use fine wire electrodes, have been explored for lower-limb motor relearning (73, 74, 75). In a single-blinded RCT, chronic stroke survivors receiving gait training with percutaneous NMES demonstrated significant improvements in gait components as measured by the Tinetti Gait scale and knee flexion coordination relative to controls who did not receive NMES (76).
Summary and Future Directions
Repetitive movement therapy, mediated by NMES, has the potential to facilitate motor relearning via both cortical and spinal mechanisms. The necessary prerequisites for NMES-mediated motor relearning include repetition, novelty of activity, concurrent volitional effort, and high functional content. Although earlier studies suggested that cyclic and EMG-mediated NMES reduces motor impairment, more recent data, especially among chronic stroke survivors, raise doubts regarding their therapeutic benefits. These approaches may be efficacious among acute stroke survivors, but there are insufficient data to confirm this. While the efficacy of cyclic
and EMG-mediated NMES in facilitating motor relearning remains uncertain, the implementation of neuroprostheses will likely have significant clinical impact due to the higher functional content.
and EMG-mediated NMES in facilitating motor relearning remains uncertain, the implementation of neuroprostheses will likely have significant clinical impact due to the higher functional content.
SPASTICITY
For over a century, researchers have attempted to quantitate the effect of ES on spasticity. Duchenne (5), in 1871, noted that stimulating the muscle antagonist to a spastic muscle resulted in a relaxation of the spastic muscle. In 1952, Levine et al. (77,78) published two articles on the inhibitory effect of ES on the spasticity of the antagonist muscles. More recently, Dewald et al. (79) has studied the effects of 10 minutes of low-intensity skin stimulation applied over the spastic biceps muscle of stroke survivors. In seven of nine subjects, ES resulted in both a reduction in peak reflex torque responses in elbow flexors and extensors that lasted for 3 minutes and a significant increase in mean threshold angle for the onset of reflex torque such that a greater angular torque was required to initiate the stretch reflex response. The authors suggested that the changes in reflex torque may reflect synaptic plasticity of spinal circuitry outside the stretch reflex loop.
The effects of TENS on spasticity in patients with various upper motor neuron (UMN) dysfunction have been described. TENS in spastic hemiparesis is associated with an increase in soleus H reflex latencies (80,81) and F-wave latencies (81), decrease in H/M and F/M ratios (81), and decrease in EMG co-contraction ratios (57). Clinical evidence of spasticity reduction associated with TENS applications in stroke include decrease in Modified Ashworth Scores (82), magnitude of plantar flexion stretch reflex (57), and resistive ankle torques (83). Underlying mechanisms for spasticity reduction secondary to TENS have been proposed and include both an enhancement in presynaptic inhibition of the spastic plantarflexor and a “disinhibition” of descending voluntary commands to the paretic dorsiflexor motor neurons (57). A sustained therapeutic effect of TENS on spasticity has not been demonstrated (35).
The effect of TENS on spasticity may be related to stimulation parameters. In a study of 32 patients with spasticity of spinal origin, high frequency (100 Hz) but not low frequency (2 Hz) stimulation was effective for the short-term amelioration of spasticity (84). The antispastic effect induced by high-frequency ES was partially reversed by a high dose of naloxone, indicating that the effect may be mediated in part by endogenous opioids.
Clinical evidence of a therapeutic modulation of spasticity associated with NMES is equivocal. Early case series suggested short-term benefits of NMES on spasticity in hemiparesis (85) and spinal cord injury (SCI) (86,87). Stimulation of the tibialis anterior (TA) muscle, prior to a mechanical stretch of the soleus muscle, has been shown to inhibit the stretch reflex of the soleus (88). In a study of spastic hemiparesis, subjects treated for 1 month with lower extremity ES showed a trend toward reduced spasticity based on Fmax/Mmax ratio, H-reflex latencies, and H-reflex recovery curves (89). As compared to a Bobath inhibitory program, a program of NMES of the dorsiflexor muscles resulted in an increase in passive ankle range of motion and reduction in modified Ashworth score (90). Other researchers have suggested that interaction of electrocutaneous stimulation with an impaired human motor control system may result in unstable reflex loops and cause excessive spastic reactions. In a study of SCI, onset times of spastic activity during an electrically elicited dorsiflexion contraction were noted to shorten with increased stimulation frequency though a stimulation burst did have a spasticity reduction effect on a subsequent burst, suggesting a potential short-term therapeutic effect of stimulation (91).
A therapeutic effect of upper (92) and lower extremity neuroprostheses on spasticity has been suggested. Several studies (93, 94, 95) have demonstrated that a neuroprosthesis can effectively open a spastic hemiparetic hand; however, adjustments of stimulation intensities and conditions of upper extremity positioning were necessary for maximal functional response. Short- (96) and longer-term (97) applications of PNS-assisted ambulation have been associated with a decrease in amplitude of the Achilles tendon reflex (96), and a decrease in the tonic activity of the TA and triceps surae muscles (97).
The efficacy of adjuvant ES combined with botulinum toxin A for treatment of spasticity has been proposed (98, 99, 100, 101), based on animal studies demonstrating enhanced toxin uptake and accelerated onset of paralytic effect by ES. Frasson et al. (99) reported that low-frequency ES (4 Hz), but not high frequency ES (25 Hz) accelerates the effect of botulinum toxin on neuromuscular blockade. In two small controlled studies, Botulinum toxin combined with NMES reduced spasticity in both the upper (102) and lower (103) extremities. However, a recent single-blinded study found no difference in efficacy of low-dose Botulinum toxin (100 U) plus short-term ES versus high-dose Botulinum toxin (400 U) to treat equinovarus spasticity (104).
In summary, there is evidence of short-term electrophysiologic effects of TENS on spasticity in patients with various UMN disorders. The functional relevance of short-term decreases in spasticity secondary to TENS is unclear; long-term modulation of spasticity secondary to TENS has not been established. A therapeutic effect of NMES on spasticity has not been established; variable outcomes may be influenced by stimulation parameters, application methods, quantification measures, and etiology of UMN dysfunction. There is evidence that NMES may enhance the antispasticity effect of Botulinum toxin; however, the superiority of NMES to other adjuvant interventions has not been established.
BONE DENSITY
The physiologic alterations of bone associated with immobility in the neurologically impaired patient are well-described. Following SCI, weight-bearing trabecular-rich sites such as the distal femur and proximal tibia show the greatest amount of
demineralization. Pathologic fractures following minor trauma can be caused by reduced bone mass in association with modified bone matrix composition (105,106). Capacitively coupled electrical fields have been shown to prevent and reverse osteoporosis in animal studies (107, 108, 109, 110, 111). Various applications of ES have been shown to stimulate osteogenesis (112), positively affect bone mineral density (BMD) (113,114), and enhance fracture healing (115) in animal models.
demineralization. Pathologic fractures following minor trauma can be caused by reduced bone mass in association with modified bone matrix composition (105,106). Capacitively coupled electrical fields have been shown to prevent and reverse osteoporosis in animal studies (107, 108, 109, 110, 111). Various applications of ES have been shown to stimulate osteogenesis (112), positively affect bone mineral density (BMD) (113,114), and enhance fracture healing (115) in animal models.
Studies have described variable short-term effects of NMES (116) and FES-cycling (117, 118, 119, 120, 121) programs on bone density in SCI. However, several studies have found site-specific effects of FES-cycling on neurogenic osteopenia. In a controlled study (122), 6 months of FES-cycling resulted in a partial reversal of BMD loss in the distal femur and proximal tibia in SCI; however, the effect was not sustained 6 months after completion of the FES-cycling program. Belanger et al. (123) measured the BMD of the distal femur, proximal tibia, and mid-tibia in SCI following a 6-month FES-cycling program. At baseline, the BMD of SCI subjects was lower than that of controls. After training, the distal femur and proximal tibia had recovered approximately 30% of the bone loss. Shields and Dudley-Javoroski (124) applied unilateral NMES to the plantar-flexor muscles in SCI using each subject as his own control. A 2-year training program resulted in a 31% higher distal tibia trabecular BMD in trained limbs as compared to untrained limbs (Fig. 71-2).
In summary, the literature supports the use of ES, in the form of lower extremity FES-cycling, to enhance bone mineral density at the distal femur and proximal tibia in SCI. However, the therapeutic effects on BMD are short-term and have not been shown to persist beyond the period of the FES-cycling intervention. The clinical efficacy of ES to prevent osteopenia, enhance BMD, or prevent fractures in non-SCI UMN paralysis has not been established.
FATIGUE, MUSCLE ATROPHY, AND STRENGTHENING
NMES has been demonstrated to have therapeutic benefit for muscle weakness and atrophy following central nervous system injury. Loss of muscle mass may occur as a result of spinal motoneuron injury resulting in denervation atrophy or central pathway injury resulting in disuse atrophy. Muscle fiber alterations at the cellular level form the basis of present understanding of NMES-associated muscle strength enhancement. Skeletal muscle contains type I (slow-twitch) fibers and type II (fast-twitch) fibers. Type I fibers are fatigue-resistant with high oxidative capacity. Type II fibers have high glycolytic capacity with the ability to metabolize adenosine triphosphate (ATP) more quickly and are thus associated with short, forceful contractions. NMES reverses the transformation of type I fibers to type II fibers, commonly seen following an UMN injury (125). In an animal model (126), changes in fiber population and myosin light chain patterns, at the level of the mitochondria and sarcoplasmic reticulum, are associated with intermittent or continuous stimulation. Ljubicic et al. (127) noted numerous biochemical and physiological adaptations induced by chronic low-frequency ES in an animal model including: alterations of calcium dynamics and myofibrillar proteins, changes in mitochondrial enzymes, myoglobin, and induction of angiogenesis. Training-induced changes in fatigue resistance seem to arise, in part, from an improved oxidative capacity. In a controlled study that compared selected morphological and metabolic properties of single fibers from the TA (128) SCI subjects who received 6 months of ES increased both the proportion of type I fibers and activity of succinate dehydrogenase (SDH) in both fiber types. Muscle fatigue has also been correlated to the level of SDH activity (129). In a study of quadriceps fiber characteristics in SCI, 12-weeks of low-frequency ES increased the SDH activity by 76% though fiber diameter and myosin heavy chain (MHC) expression remained unchanged. The specific effects of NMES-facilitated exercise on the motor unit including muscle fiber transformation as an adaptive response have been summarized (130,131).
NMES can play a role in preventing or reversing muscular atrophy following UMN injury. Long-term FES training programs post-SCI can prevent lower extremity muscle atrophy (132), increase cross-sectional muscle area (133,134), and preserve the physiological properties of specific muscles (peak torque, fatigue index, torque-time integral, and contractile speed) (124). In a study by Kern (135), an increase in quadriceps muscle fiber diameter (mean 59%) and muscle cross-sectional area (mean 30%) was noted following an 8-month training period with FES paraplegic subjects. Dupont Salter et al. (136) investigated the effects of four different stimulation paradigms on disuse atrophy produced in the TA, lateral gastrocnemius, and soleus muscles of paralyzed rats. Lower frequency stimulation (2 Hz) applied to the soleus resulted in 10% mean atrophy as compared to 26% atrophy at 10 Hz and 32% atrophy in unstimulated, paralyzed controls. The authors proposed possible mechanisms for the selective effect
of low-frequency ES on muscle atrophy including variable muscle loading conditions, effects of both animal gender and anesthesia on hormone levels (cortisol, testosterone, growth hormone), and conversion of fast to slow muscle fibers associated with higher-frequency stimulation-induced increases in basal cytosolic calcium levels.
of low-frequency ES on muscle atrophy including variable muscle loading conditions, effects of both animal gender and anesthesia on hormone levels (cortisol, testosterone, growth hormone), and conversion of fast to slow muscle fibers associated with higher-frequency stimulation-induced increases in basal cytosolic calcium levels.
A limited number of studies have evaluated the efficacy of NMES on muscle strength when applied to the upper extremity. Peckham et al. (137) demonstrated an increase in contractile force resulting in functional gain with the application of ES to the forearm finger flexors of ten quadriplegic patients. However, Seeger et al. (138) studied the effect of FES-assisted exercise versus isotonic exercises and found no increase in maximum voluntary upper extremity force in SCI. In separate studies, NMES applied to the wrist extensors in hemiplegia has also been demonstrated to be associated with higher isometric wrist extension torques (37), greater isometric finger extension (44), and improved grip speed (139).
The ability of NMES, used in various applications, to enhance the strength of specific lower extremity muscles post-SCI has been demonstrated. Cyclic NMES to the quadriceps has been shown to enhance quadriceps muscle strength (123,140). Lima (141) compared NMES to isotonic exercises of the knee and found that flexor torque, though not extensor torque, was increased in the NMES group. Similarly, FES-cycling programs (142,143) and lower-extremity FES-exercise programs (144) have been shown to be effective in increasing the muscle strength in SCI. Sloan et al. (145) reported improvements in voluntary isometric strength, stimulated isometric strength and stimulated isometric endurance of the quadriceps, muscle grading of the quadriceps and biceps femoris and the cross-sectional areas of the quadriceps and total thigh muscle associated with a 3-month FES-cycling program in SCI. Guidelines for FES training in SCI were proposed by Petrofsky et al. (146) who studied 90 paraplegic patients and determined that the optimal protocol for FES-isokinetic exercise of the quadriceps was a three time per week regimen for 30 min/day with equal alternating periods of work and rest. Kern et al. (147) determined that the striated appearance of normal skeletal fibers may be maintained up to 20 years post-SCI and thus proposed that there was no upper limit for the initiation of a training program based on FES in SCI. Several reviews outline the use of NMES to increase strength and endurance in the SCI (148, 149, 150), stroke (151), and able-bodied patient (152) populations.
In summary, on a cellular level, NMES has the ability to reverse the transformation of type I fibers to type II fibers that is commonly seen following an UMN injury. NMES can play a preventative role in minimizing the amount of muscular atrophy seen following an UMN injury. The ability of cyclic NMES or FES-cycling to reverse muscle atrophy and enhance lower extremity muscle strength in SCI is supported by the literature and accepted in clinical SCI care. While NMES may be clinically used to prevent muscle atrophy and enhance strength in other UMN disorders including stroke, the clinical and functional relevance of resultant strength changes has not been established.
TISSUE OXYGENATION AND WOUND HEALING
Early studies (153,154) proposed the therapeutic benefit of ES in the treatment of soft tissue injury and wound healing. Subsequent studies have demonstrated that ES affects wound healing by both a direct local tissue effect at the cellular level and via an enhancement of angiogenesis, tissue perfusion, and oxygenation (155, 156, 157). Certain chemotaxic factors found in wound substrates contribute to tissue repair processes by attracting cells, which carry positive or negative charges, into the wound environment. ES can facilitate the induction of directional cell migration (i.e., electrotaxis) (158) of neutrophils, macrophages, epidermal cells, and fibroblasts into the wound. These cells accelerate wound healing through their specific cellular roles in autolysis, cellular protein synthesis, mitotic cell division, and anti-inflammatory activities (159,160). In a recent review, Kloth (155) presented the relationship between endogenous bioelectric currents and wound repair, and reviewed clinical research applications of ES on wound healing.
ES induces angiogenesis to enhance tissue perfusion and oxygenation. In a study by Zhao et al. (161), applied electric fields of small physiological magnitude directly stimulated vascular endothelial growth factor (VEGF) production by endothelial cells in vitro. They proposed that endogenous electric fields (EFs) might play a role in angiogenesis in vivo by stimulating the VEGF receptor signaling pathway to induce key preangiogenic responses. Other animal studies have demonstrated ES-induced changes including increased capillaryto-fiber ratios, blood perfusion, transformation of muscle fiber types from mixed (oxidative and glycolytic) to primarily oxidative fibers, and improved muscle fatigue (162, 163
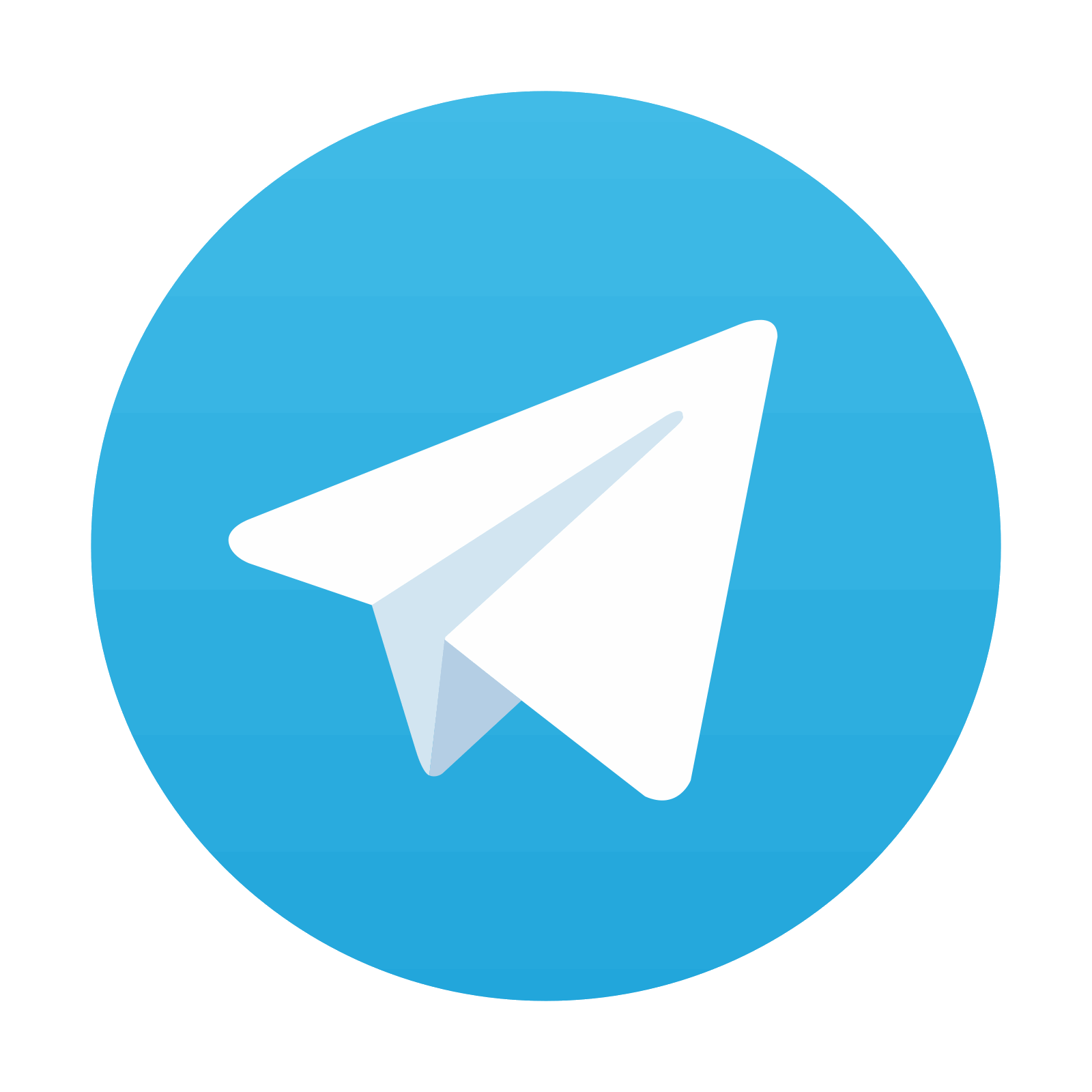
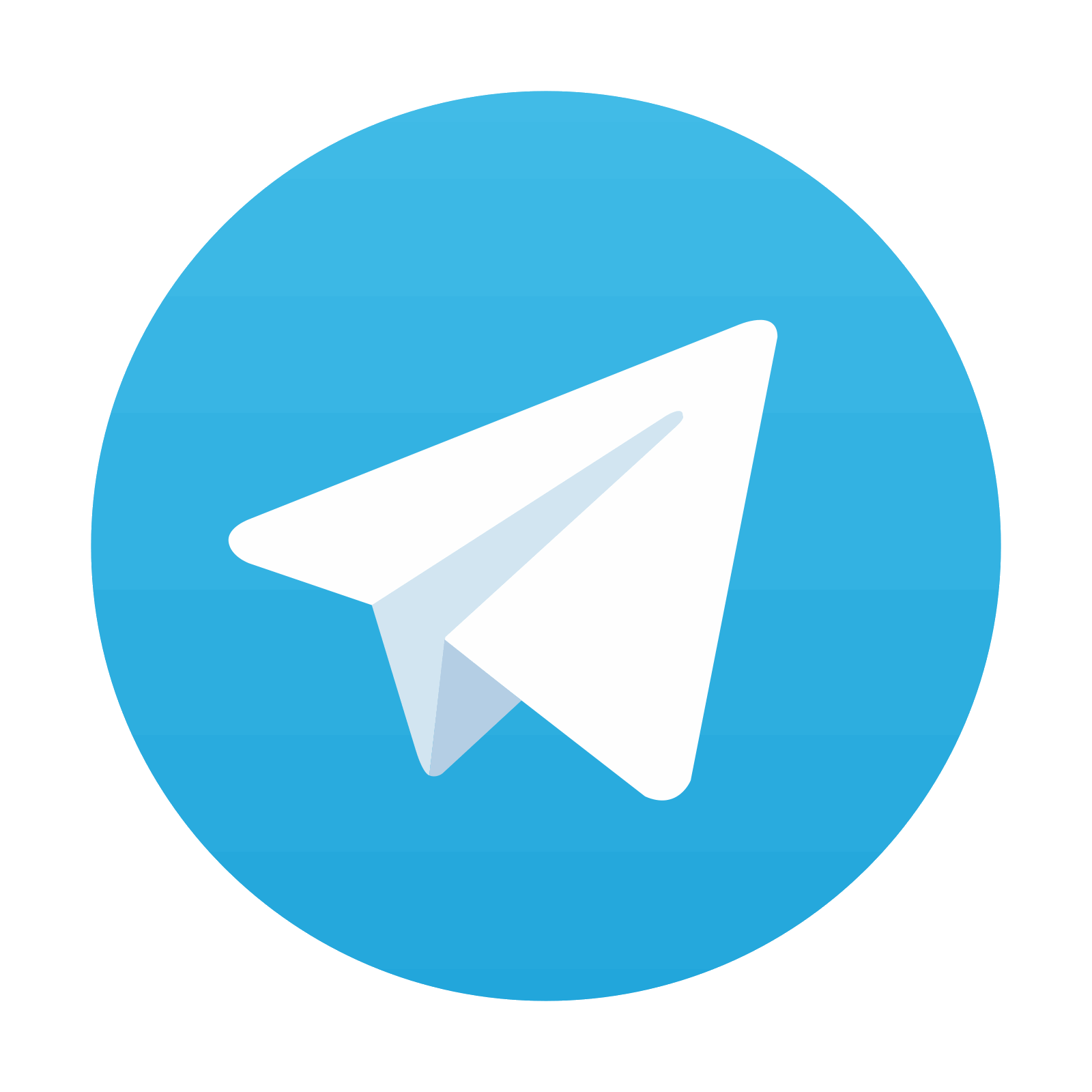
Stay updated, free articles. Join our Telegram channel
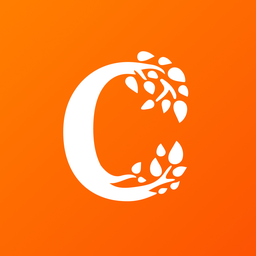
Full access? Get Clinical Tree
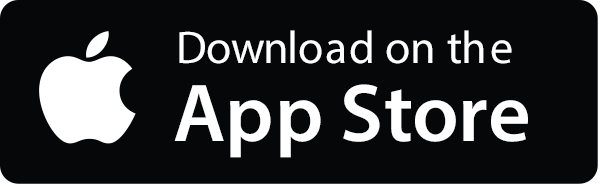
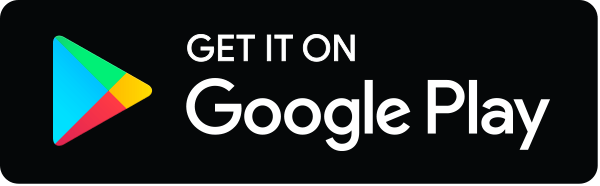