Mutations in the dystrophin gene cause Duchenne and Becker muscular dystrophy in humans and syndromes in mice, dogs, and cats. Affected humans and dogs have progressive disease that leads primarily to muscle atrophy. Mdx mice progress through an initial phase of muscle hypertrophy followed by atrophy. Cats have persistent muscle hypertrophy. Hypertrophy in humans has been attributed to deposition of fat and connective tissue (pseudohypertrophy). Increased muscle mass (true hypertrophy) has been documented in animal models. Muscle hypertrophy can exaggerate postural instability and joint contractures. Deleterious consequences of muscle hypertrophy should be considered when developing treatments for muscular dystrophy.
By the age of 3 years, his mother noted that his lower extremities grew in volume. Her attention was first drawn to this enlargement of his calves which entered his stockings with difficulty. —Duchenne’s description of his first patient, Joseph Sarrazin, as cited by Tyler
Mutations in the human dystrophin gene cause 2 clinical phenotypes, Duchenne muscular dystrophy (DMD) and Becker muscular dystrophy (BMD), distinguished principally based on the age at which patients lose the ability to ambulate. Boys with DMD become wheelchair users before 14 years of age, whereas those with BMD walk beyond age 16 years. The basis for this clinical distinction can largely be traced to the fact that DMD mutations result in the loss of the mRNA reading frame, virtually eliminating dystrophin protein production, and BMD mutations preserve the reading frame, allowing production of a partially functional protein. Muscles of patients with both forms express variable pathologic changes that generally lead to profound muscle atrophy. In contrast, some muscles, most notably the gastrocnemius, enlarge. Although hypertrophy has typically been attributed to deposition of fat and connective tissue, so-called pseudohypertrophy, imaging studies have shown true hypertrophy in some individuals.
There are 3 mammalian models in which spontaneous dystrophin gene mutations lead to distinct phenotypes of muscular dystrophy: the mdx mouse, golden retriever muscular dystrophy (GRMD) dog, and feline hypertrophic muscular dystrophy (FHMD) cat. The GRMD model most closely mirrors DMD at multiple levels, including progressive disease that leads primarily to muscle atrophy. The mdx mouse progresses through an initial phase of muscle hypertrophy followed in old age by atrophy. In contrast, the FHMD cat has persistent muscle hypertrophy. The role of true hypertrophy has been more broadly accepted in these models, with less attention paid to contributions made by fat and connective tissue in muscle enlargement.
In principle, relative muscle sparing or hypertrophy could be either beneficial or detrimental. The benefits are obvious because preservation of strength should enhance motor function in activities ranging from ambulation to breathing. Harmful effects are less clear and relate to the potential for muscle hypertrophy to exacerbate contractures and postural instability. Additional deleterious consequences occur due to difficulties in eating because of glossal hypertrophy and regurgitation resulting from either esophageal hypomotility or obstruction at the level of the hypertrophied diaphragm. Whether the hypertrophy results from an actual increase in muscle mass or fat and connective tissue, studies directed at defining underlying mechanisms could provide insight into the pathogenesis of dystrophin deficiency and inform treatment development.
Variable muscle involvement and hypertrophy
Effects of dystrophin deficiency vary among species, individuals, and muscles. Reasons for phenotypic variation are poorly understood and raise questions about primary versus secondary effects of dystrophin deficiency.
DMD and BMD
Extensor muscles that undergo eccentric muscle contraction, such as the quadriceps femoris, are particularly vulnerable in DMD. In contrast, the extraocular muscles are largely spared, and other muscles undergo striking paradoxical hypertrophy. Although attention has focused on gastrocnemius (calf) enlargement ( Fig. 1 ), hypertrophy occurs in many other muscles. Presumptive cases of DMD characterized by hypertrophy of the calves, deltoid, and infraspinatus muscles were seen in Italy and England as early as the 1830s well before Duchenne’s classic account. In a 1995 review of 84 patients with DMD from India, 94% had calf enlargement, followed by the infraspinatus (88%), deltoid (52%), and tibialis anterior (40%). The selective muscular involvement extended to different heads of the deltoid and quadriceps, which showed concomitant atrophy and hypertrophy. Calf hypertrophy was evident on physical examination in 20 of 26 (77%) BMD cases in another study. These patients were further studied with computed tomography to determine the pattern and course of muscle involvement. Hypertrophy was seen in the calves (42%), sartorius (42%), gracilis (42%), adductor longus (38%), semitendinosus (19%), and rectus femoris (11%). Patients with DMD and/or BMD have also been shown to have hypertrophy of the tongue (macroglossia), diaphragm, and hypothenar (palm) muscles, among others.
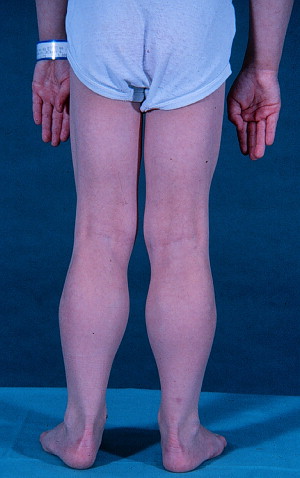
Dating to Duchenne’s monograph, muscle hypertrophy in DMD and BMD has been attributed to deposition of fat and connective tissue, giving rise to the term pseudohypertrophic muscular paralysis. Indeed, histopathologic studies have documented fibrosis and fatty change in the calves and other hypertrophied muscles. Walton speculated that true hypertrophy also contributes to muscle enlargement, perhaps occurring early in the disease course, followed by pseudohypertrophy. However, because of inherent limitations of sequential muscle sampling in human patients, the time course and relative roles of true hypertrophy and pseudohypertrophy have remained unsettled. Specialized imaging techniques have complemented and, in some cases, essentially replaced histopathologic evaluation of muscle biopsy samples. Increasingly, use of these techniques has documented features consistent with a true increase in contractile mass. In one such study, 10 of 16 patients with BMD had calf enlargement on ultrasonography, and 9 of them were judged to have true hypertrophy. Another study, cited earlier, found that enlarged muscles visualized with computed tomography were often rounded and had normal densities, suggesting true hypertrophy. A further paper, in which magnetic resonance imaging (MRI) was evaluated, showed that the gracilis and sartorius muscles were relatively spared and/or hypertrophied in 10 patients with DMD. We are particularly intrigued by the relative sparing of the sartorius muscle ( Fig. 2 ) because of our own studies of cranial sartorius hypertrophy in GRMD (see later).
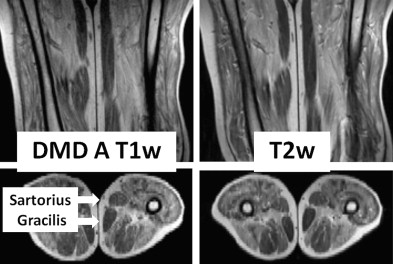
Ideally, imaging and histopathologic results should be correlated to define the relative contributions that fat, fibrous connective tissue, and myofiber hyperplasia or hypertrophy make to muscle enlargement. Few such studies have been completed in DMD. In one paper that correlated computed tomography and histochemistry findings, apparent true hypertrophy of the gastrocnemius in a 7-year-old boy with DMD was judged to be caused by myofiber hyperplasia rather than hypertrophy. With this said, hypertrophied myofibers are also seen early in the disease and persist throughout life.
The mdx Mouse
More extensive pathologic studies can be done with animal models, potentially allowing better definition of mechanisms contributing to differential muscle involvement. Just as in DMD, muscles are variably affected in the mdx mouse, ranging from the unaffected extraocular muscles to the severely involved diaphragm. Limb muscles undergo dramatic necrosis at 3 to 4 weeks of age followed by robust regeneration and muscle hypertrophy. Changes vary among muscles, with the predominantly slow twitch soleus being more involved at 3 to 4 weeks and the fast twitch extensor digitorum longus at 32 weeks and beyond. Muscle hypertrophy causes mdx mice to be larger than normal between about 10 and 40 weeks of age, after which they lose weight in concert with a loss of muscle mass. Reflecting the early strong regenerative response, mdx mice have increased numbers of small regenerating fibers with central nuclei at 3 weeks of age. The percentage of larger regenerated myofibers increases with age, approaching 70% and 90% in the soleus and extensor digitorum longus, respectively, by 26 weeks. Because populations of both small and large fibers occur concomitantly, mean fiber diameter of mdx mice typically is normal. The concomitant occurrence of large and small fibers suggests that both hyperplasia and hypertrophy contribute to muscle hypertrophy.
Unlike DMD, mdx mice have minimal fatty change and fibrosis, indirectly implicating true hypertrophy as the cause for muscle enlargement. The time course of pathologic lesions in the mdx mouse leads to corresponding functional changes, with affected mice being weaker at 2 to 4 weeks and then recovering. Absolute tension generated by mdx muscles is typically higher by 8 to 16 weeks of age. When corrected for cross-sectional area, isomeric soleus muscle force of younger (≤100 days) mdx mice was lower than normal, whereas values for older (≥100 days) mdx mice were higher. Coulton and colleagues commented that, “when mdx mice lose muscle fibers by necrosis, they do not just replace them with equally efficient new muscle, but with heavier, stronger, muscles than the wild-type.” Typical of this pattern and consistent with our own findings in the GRMD dog (see later), isometric tension generated by the tibialis anterior is considerably lower in mdx mice at 3 to 4 weeks but exceeds normal values by 8 to 16 weeks. Pathologic changes in the tibialis anterior also occur earlier than those in the soleus and gastrocnemius muscles. Taken together, these mdx mouse data show clear evidence that early necrosis leads to a dramatic regenerative response that can lead to functional hypertrophy.
Contraction kinetics in mdx mice also differ from normal. Relaxation times were increased in the soleus, independent of age, and in the tibialis anterior at 3 to 4 weeks but not at later ages. Although twitch-tetany ratios were decreased compared with normal in the soleus of variably aged mdx mice, a reverse relationship was seen in the tibialis anterior at 7 to 8 weeks. Relaxation times of the mdx tibialis anterior were also increased at 3 to 4 weeks, but values normalized in older mice.
FHMD
Male cats with weakness, histologic features typical of muscular dystrophy, and gross and histologic evidence of muscle hypertrophy were characterized before the discovery of dystrophin. Subsequently, an analogous syndrome was reported in cats with dystrophin deficiency. One of these FHMD cats was shown to have a dystrophin gene deletion that included the skeletal muscle and neuronal Purkinje cell promoters and first exons. The most striking clinical feature in all these cats was gross hypertrophy of their axial and appendicular muscles, as well as the tongue, diaphragm, and esophageal muscularis. In one study, 4 muscles (biceps brachii, cranial tibialis, gastrocnemius, and diaphragm) from 2 FHMD cats weighed twice as much as those from comparably sized normal cats. Physical evidence of hypertrophy has been noted as early as 3 months and seems to become more pronounced with age. As an example, the circumference of the neck in one cat increased from 28 to 33 cm between 14 and 25 months of age. On microscopic examination, FHMD cats have myofiber size variation, with increased populations of both small regenerating and hypertrophied myofibers and an overall mean myofiber diameter in the normal range. In keeping with progressive hypertrophy, the mean myofiber diameter of FHMD cats increased more so than that of normal cats between 3 to 4 and 6 to 9 months of age. In addition, there was myofiber necrosis and splitting and both gross and individual myofiber mineralization. The relative absence of fibrosis in FHMD cats up to 2 years of age suggests that the muscle enlargement is most likely because of true hypertrophy.
GRMD Dog
As with DMD and the mdx mouse, the extraocular muscles are largely spared in GRMD. Other muscles become involved as a function of age and usage. Muscles that are used heavily in utero and early in life, such as the tongue, diaphragm, and limb flexors, are acutely necrotic during the neonatal period. Extensor muscles demonstrate a more delayed pattern of involvement, reflecting their greater use in weight bearing. As with the mdx mouse, muscles that undergo early necrosis may then regenerate and even hypertrophy. In one of the original GRMD dogs studied by our group, the hamstrings, tongue, diaphragmatic crura, and esophageal muscularis were enlarged.
A further study in our laboratory showed that most GRMD pelvic limb muscles atrophy, whereas the caudal and cranial sartorius and popliteus hypertrophy. Cranial sartorius muscle weights were corrected for body weight and endomysial space to determine true muscle weights (g/kg) in 3 GRMD age groups (4–10, 13–26, and 33–66 months) and grouped normal dogs (6–20 months) ( Table 1 ). Corrected GRMD weights in the younger dogs were greater than those of normal dogs, indicating that the cranial sartorius undergoes initial true muscle hypertrophy. Values of both older groups were less than those of the younger dogs, suggesting that the cranial sartorius muscle atrophies over time, with an associated increase in the endomysial space because of deposition of fat and connective tissue.
Pathologic Lesion | Normal (6–20 mo; n = 12) | GRMD | ||
---|---|---|---|---|
Group 1 | Group 2 | Group 3 | ||
4–10 mo; n = 15 | 13–26 mo; n = 4 | 33–66 mo; n = 4 | ||
Corrected muscle weight (g/kg) a | 1.3075 ± 0.2079 | 3.0573 ± 0.7635 | 2.4725 ± 0.7556 | 1.4650 ± 0.6575 |
Percentage of endomysial space b | 2.8083 ± 1.3468 | 27.1857 ± 15.3869 | 44.1275 ± 14.6462 | 58.9100 ± 14.2060 |
True muscle weight (g/kg) c | 1.2699 ± 0.1966 | 2.2063 ± 0.6884 | 1.3758 ± 0.5078 | 0.5720 ± 0.2423 |
Mean fiber diameter (μm) d | 42.1658 ± 4.3542 | 57.4980 ± 11.7419 | 63.1295 ± 13.3033 | 47.0850 ± 17.7029 |
a Normal<GRMD, Group 1 ( P <.05); GRMD Group 1>GRMD Group 3 ( P <.05).
b Normal<GRMD Groups 1–3 (all P <.05).
c Normal<GRMD, Group 1 ( P <.001); GRMD Group 1>GRMD Groups 2 ( P <.05) and 3 ( P <.001).
d Normal<GRMD Groups 1 and 2; P <.01 for Group 1 and P <.05 for Group 2.
Our studies of force/torque generated by individual and grouped GRMD muscles are in keeping with these pathologic data and findings from mdx mice. We initially evaluated tension generated by the peroneus longus muscle. Absolute twitch tension and both muscle- and body-weight-corrected twitch tension in GRMD dogs were low compared with normal littermates at 3 months of age. Tetanic tension was affected similarly. However, although absolute values were still reduced at 6 months, twitch and tetanic tension corrected for either muscle or body weight was not statistically different ( Fig. 3 ), suggesting that the peroneus longus recovers from an initial period of necrosis. Moving forward, we have primarily evaluated force/torque generated by tarsal joint flexors (including the cranial tibialis and peroneus longus) and extensors (including the gastrocnemius and superficial digital flexor that is analogous to the soleus). For these measurements, the peroneal and tibial nerves are stimulated percutaneously so that the paw pulls (peroneal nerve, flexion) or pushes against (tibial nerve, extension) a lever interfaced with a force transducer. In our initial study, force values were measured at 3, 4.5, 6, and 12 months of age. While absolute and body-weight-corrected GRMD twitch and tetanic force values were lower than normal at all ages, tarsal flexion and extension were differentially affected ( Fig. 4 ). Flexion values were especially low at 3 months, whereas extension was affected more at later ages.
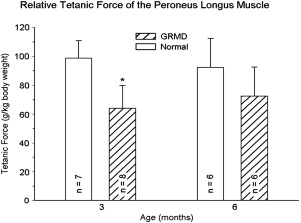
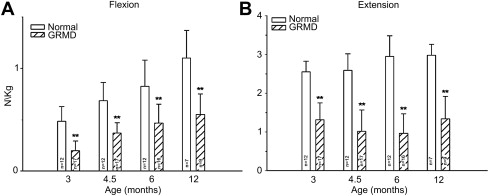
We have used tetanic tarsal joint force measurements to evaluate effects of prednisone (2 mg/kg) given to GRMD dogs for a 4-month period beginning at 2 months of age. Tarsal extension force increased in treated versus control GRMD dogs, whereas flexion paradoxically decreased. In light of our force studies, we assumed that the paradoxical decline in flexion occurred because prednisone attenuated early necrosis in muscles such as the peroneus longus and cranial tibialis that would have otherwise led to functional hypertrophy.
Contraction kinetics in GRMD dogs for both the peroneus longus and grouped tarsal joint flexors and extensors also differed from normal. Post-tetanic potentiation for the peroneus longus was more pronounced in GRMD versus normal dogs at both 3 and 6 months. Twitch contraction and relaxation times were dramatically prolonged, and there was concomitant sustained electrical activity at or before 6 months of age in some severely affected dogs. For the grouped muscles, the twitch-tetany ratio was generally lower, post-tetanic potentiation for flexion values was less marked, and extension relaxation and contraction times were longer. As discussed further later, prolonged relaxation times with underlying sustained electrical activity and more generalized complex repetitive discharges could play a role in muscle hypertrophy.
Postural instability and contractures
Comparative aspects of gait abnormalities, postural changes, and joint contractures in humans and animal models must be interpreted in light of fundamental differences in conformation, some of which arise because of quadrupedal versus bipedal locomotion. As an example, the line of the pelvis from the tuber ischium to the wing of the ilium extends in a vertical plane, perpendicular to the walking surface, in humans but is oriented more horizontally, largely parallel to the ground, in quadrupeds. Thus, changes in pelvic orientation innately differ among patients with DMD, mdx mice, and GRMD dogs. In some cases, muscle weakness in DMD causes the posture to shift toward one normally assumed by quadrupeds and vice versa. The pelvis tilts anteriorly toward a more horizontal position to maintain postural stability in DMD but shifts toward a vertical plane in GRMD dogs (see later). Another factor relates to whether the leg/limb stance is plantigrade (humans and mice) or digitigrade (dogs). Humans and mice walk on their phalanges, carpal, and tarsal bones, whereas dogs normally walk only on their distal and intermediate phalanges (digits). With neuromuscular diseases, in general, dogs become more plantigrade in the pelvic limbs, adopting a stance more in keeping with that normally used by humans. A third stance, termed unguligrade, involves walking only on the tip of the distal digit. The fact that horses have this posture explains use of the term equinus in DMD patients who toe walk.
DMD and BMD
Most DMD natural history studies include measurements of muscle strength, joint contractures, and timed function tests. Results from these tests are used to track disease progression and offer insight on clinical milestones, such as the loss of ambulation and the need for ventilatory support. Contracture and muscle strength scores generally correlate, deteriorate synchronously over time, and contribute mutually to postural instability. As discussed later, unequal muscle weakness in DMD precipitates a vicious cycle that can lead to debilitating contractures and loss of ambulation ( Fig. 5 ).
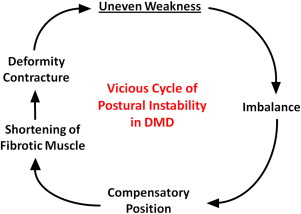
Generally, contractures are caused by inactivity and restricted motion of the affected joint, with a subsequent increase of collagen cross-links in periarticular connective tissue. Major causes include forced joint immobilization to stabilize fractures, spasticity associated with upper motor neuron lesions, and primary neuromuscular diseases. Joint contractures occur more commonly in DMD than other neuromuscular diseases and have long been recognized as a major factor in disease morbidity. In one review that included 43 patients with DMD, the ankle (34/43), knee (29/43), hip (29/43), and elbow (28/43) were affected most frequently. The ankle was also most commonly affected in BMD, occurring in 4 of 7 patients. Despite the prominent role that joint contractures play in these dystrophies, causative mechanisms are not fully understood. Underlying abnormal positioning presumably occurs because of an imbalance of forces acting on the joint, because diseased muscles are either disproportionately weakened or shortened by fibrosis. Relevant to this review, several studies have assessed the proportional strength of agonist and antagonist muscles operating at joints of patients with DMD. Some studies have concluded that muscular imbalance contributes to contractures, whereas another found no such association. Those finding a relationship noted a strong negative correlation between extensor muscle weakness and flexor contracture severity in DMD. As opposing extensor muscles weakened, flexor contractures worsened. Disproportionate flexor muscle hypertrophy would logically exaggerate this process. In a somewhat similar vein, contractures occurring because of spasticity also are magnified by enhanced flexor muscle activity.
Postural instability and leg contractures are of special interest in DMD because of their role in the loss of ambulation. The relative sequence and proportional involvement of flexor and extensor muscles is critical. Early weakness of the hip (gluteus maximus) and knee (quadriceps femoris) extensors necessitates postural changes to maintain ambulation. Increased anterior pelvic tilt and lumbar lordosis are adopted to shift the center of gravity forward of the knee and behind the hip, respectively. Johnson suggested that this posture allows passive ligamentous stabilization of these joints. Of comparative interest, a somewhat analogous system of ligamentous support (stay apparatus) allows horses to remain standing while sleeping. Relative preservation of hip (including the sartorius) and knee (hamstrings) flexors in DMD creates destabilizing torque forces and also contributes to contractures at both levels. Toe walking is adopted to stabilize the knee and later plays a role in the development of ankle equinus. Plantar flexor contractures associated with equinus are aggravated by unbalanced muscle activity at the ankle, with selective weakening of the tibialis anterior and peroneus longus muscles and relative sparing of the triceps surae (collectively, the 2 heads of the gastrocnemius and soleus). One investigator characterized this as a return to the infantile digitigrade pattern of walking, perhaps in an effort to put less stress on the weakened tibialis anterior muscle. These contractures may initially have beneficial effects because tension in the gastrocnemius muscles pulls on the femoral condyles, extending the knee. Iliotibial band (hip) contractures also extend the knee, providing additional stability. However, in advanced stages, heel cord and iliotibial band tightening destabilize gait, prompting the development of various corrective surgical procedures. Scoliosis is typically a late complication of DMD, occurring in boys after they have gone into wheelchairs, with the side of convexity almost always toward the dominant hand. The developing spine is thought to become deformed by excessive unbalanced forces from the dominant extremity. Scoliosis restricts expansion of the chest and tracks closely with respiratory disease. Importantly, this biomechanical interplay in scoliosis shows that application of disproportionate (asymmetric) muscle force to the developing skeleton can cause bony maldevelopment with clinical consequences.
As discussed earlier, mechanisms contributing to preferential calf and other flexor muscle sparing or hypertrophy, starting with whether there is an actual increase in contractile tissue, are especially intriguing. If one accepts that these muscles undergo true hypertrophy in at least some patients, the timing and functionality of the muscle enlargement and whether it is playing a beneficial or detrimental role become critical. This would be particularly relevant with treatments, such as myostatin inhibition, that are intended to increase muscle mass (see later).
The mdx Mouse
Kyphosis analogous to scoliosis in DMD has been characterized in the mdx mouse. Just as with DMD, there is an association between spinal deformity and respiratory compromise. Mechanisms to account for kyphosis have not been defined, although Laws and Hoey speculated that muscle hypertrophy could contribute. In a separate proof-of-concept study, this same group evaluated the potential for intramuscular injection of antisense oligonucleotides into paraspinal muscles to ameliorate kyphosis. The treated group had less thoracic deformity than controls, but other outcome parameters did not differ between the groups. Weights of the latissimus dorsi, diaphragm, and intercostals muscles were increased in mdx mice in both treated and control mice. Force generation by these muscles was not increased, and histologic features were more in keeping with muscle degeneration and fibrosis than true hypertrophy. Thus, although not the central focus of this study, there was not a definite link between muscle hypertrophy and kyphosis.
Contractures of the tarsal (talocrural, ankle) joint have recently been characterized in the mdx mouse. As discussed earlier, both mice and humans have a plantigrade stance, so there could be analogous operative forces. In keeping with findings from DMD, mdx mice had plantar flexor contractures, evidenced by decreased dorsiflexion and range of motion. There was an associated increase in gastrocnemius wet muscle weight, and torque generated by the dorsiflexors was proportionally lower than that of the gastrocnemius muscles.
FHMD
Because of their muscle hypertrophy, FHMD cats have a stiff stilted gait with their hocks adducted. They adopt a “falling down technique” to move into lateral recumbency and are unable to turn their heads and groom. FHMD cats also do not close their mouths completely, presumably because of glossal hypertrophy, which interferes with eating and swallowing, ultimately causing dehydration and azotemia. The esophagus may have decreased contractility and can be constricted by the hypertrophied diaphragm, resulting in regurgitation. A syndrome similar to malignant hyperthermia has been characterized in several cats that died during stress or anesthesia (see discussion linking calcium homeostasis in this condition and muscle hypertrophy).
GRMD Dog
Gait and postural changes in GRMD must be considered in the context of marked phenotypic variation among affected dogs. Severely affected dogs may die within the first 10 days of life, whereas others live well into adulthood. By 6 weeks of age, GRMD dogs advance their pelvic limbs simultaneously ( bunny hopping ) and subsequently exhibit a progressively more stilted gait. Those with a severe phenotype develop a characteristic plantigrade stance between 3 and 6 months, as evidenced by hyperextension of the carpus and hyperflexion of the tarsus. Over this same period, the elbows become abducted and the hocks are adducted. Concomitantly, the pelvic limbs shift forward, as the tuber ischium of the pelvis moves ventrally and cranially. In severe cases, the line of the pelvis may be oriented in an essentially vertical plane, perpendicular to the walking surface ( Fig. 6 ). Some of these dogs lose the ability to walk and must be euthanized.
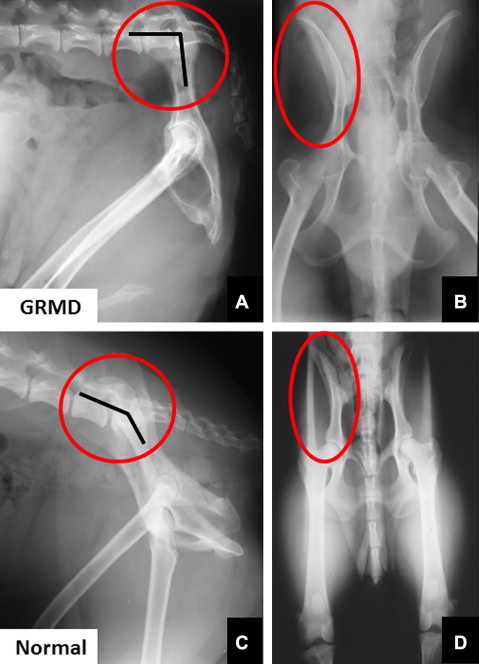
Beyond the initial 6 months of life, clinical signs in GRMD dogs tend to stabilize and further adaptive changes help to maintain postural stability. With both video gait analysis and accelerometry, GRMD dogs walk more slowly and their stride length is decreased. Accelerometry demonstrated a redistribution of power at gait, with a decrease in the craniocaudal plane and a compensatory increase mediolaterally to maintain balance. On video gait analysis, older, generally mildly affected dogs had a more upright stance, with relatively greater extension of the stifle and lesser flexion of the tarsus. This posture presumably is adopted in an effort to stabilize their stance in the face of quadriceps weakness. In our experience, GRMD dogs rarely become nonambulatory after the initial critical period of destabilization at 3 to 6 months of age. Some have a normal life span; we currently have a 12-year-old GRMD dog with a remarkably mild phenotype in our colony.
One of our earlier studies showed that 6-month-old GRMD dogs positioned in dorsal recumbency for force measurements have abnormally acute (contracted) tarsal joint angles. Other investigators have subsequently described methods to measure joint angles at maximal flexion and extension in normal dogs. We now use the method suggested by Jaegger and colleagues to measure pelvic limb joint angles and range of motion. By 6 months of age, GRMD dogs tend to have more restricted maximal flexion of the hip joint, increased maximal stifle extension, and more acute maximal tarsal flexion. To objectively characterize the cranioventral shift of the pelvis, we also measure the angle formed by 2 lines extending cranially from the tuber ischium, one drawn parallel to the lumbar spine and the other extending to the midpoint of the tuber coxae. This angle is larger in dogs with GRMD than in normal dogs at 6 months of age.
Mechanisms contributing to postural and joint angle changes in GRMD dogs have not been defined. The cranioventral pelvic shift may be an adaptive response, as affected dogs move their pelvic limbs under the torso to maintain balance. The resultant posture is similar to that achieved by boys with DMD when they shift their pelvis forward. Alternatively, unbalanced hip flexor and extensor strength might play a role. Relative preservation of the hamstring muscles in GRMD dogs could pull the tuber ischium ventrally and also contribute to decreased maximal hip flexion values. As discussed earlier, considering the role that the sartorius and iliotibial band play in hip flexor contractures in DMD, true hypertrophy of the cranial sartorius could be playing an analogous role in GRMD. In support of a relationship, we have previously shown that cranial sartorius circumference correlates negatively with tarsal joint angle in affected dogs. Still, it is unclear whether there is truly a cause-and-effect relationship. The hypertrophied cranial sartorius could actively pull the stifle joint forward, with the tarsus passively following to assume a plantigrade position. On the other hand, cranial sartorius hypertrophy and plantigrade stance might have common root causes but no direct functional relationship. In any case, cranial sartorius hypertrophy or contracture does seem to affect the developing pelvis in young GRMD dogs, as the ilial wings from which it originates flare laterally (see Fig. 6 ), presumably in response to unopposed torque. This is somewhat analogous to scoliosis resulting from unbalanced force applied by the dominant arm in boys with DMD and emphasizes the potential for disproportionate muscle size and strength to cause skeletal deformity.
Local imbalance of agonist and antagonist muscles could also be playing a role in postural changes at the tarsal and carpal joints of GRMD dogs. Consistent with findings in DMD, we have shown that GRMD extensor and flexor muscles operating at the tarsal joint are differentially affected. Flexion values are especially low at 3 months, whereas extension is affected more at later ages (see Fig. 4 ). At 6 months of age, the tarsal extension-flexion force ratio correlates positively with tarsal joint angle, which is to say that dogs with stronger extensors have larger joint angles and a less severe phenotype. We have not systematically studied joint angles in the thoracic limb and can only speculate on mechanisms involved in carpal hyperextension. It seems unlikely that a reverse pattern of muscle involvement, whereby extensor muscles are relatively preserved, is responsible. Importantly, plantigrade stance is a nonspecific clinical sign in dogs and cats with neuromuscular disease, independent of underlying cranial sartorius hypertrophy. This suggests that the pelvic limb plantigrade posture, coupled with hyperextension of the carpus (so-called palmigrade stance), may simply reflect distal muscle weakness. The pivot point would logically differ between the carpus (hyperextension) and tarsus (hyperflexion) to keep the footpads in contact with the walking surface.
Spinal curvature is not a major feature of GRMD. Lumbar kyphosis may occur in tandem with the cranioventral shift of the pelvis; lordosis can be seen more chronically. The lordotic posture could occur because of unequal application of force on the developing spine, with relative restriction of vertebral growth dorsally (posteriorly) or exaggerated growth ventrally (anteriorly). Relative preservation of the psoas major muscle in GRMD dogs might exert disproportionate force on the ventral lumbar spine, contributing to both hip flexor contractures and lordosis.
Postural instability and contractures
Comparative aspects of gait abnormalities, postural changes, and joint contractures in humans and animal models must be interpreted in light of fundamental differences in conformation, some of which arise because of quadrupedal versus bipedal locomotion. As an example, the line of the pelvis from the tuber ischium to the wing of the ilium extends in a vertical plane, perpendicular to the walking surface, in humans but is oriented more horizontally, largely parallel to the ground, in quadrupeds. Thus, changes in pelvic orientation innately differ among patients with DMD, mdx mice, and GRMD dogs. In some cases, muscle weakness in DMD causes the posture to shift toward one normally assumed by quadrupeds and vice versa. The pelvis tilts anteriorly toward a more horizontal position to maintain postural stability in DMD but shifts toward a vertical plane in GRMD dogs (see later). Another factor relates to whether the leg/limb stance is plantigrade (humans and mice) or digitigrade (dogs). Humans and mice walk on their phalanges, carpal, and tarsal bones, whereas dogs normally walk only on their distal and intermediate phalanges (digits). With neuromuscular diseases, in general, dogs become more plantigrade in the pelvic limbs, adopting a stance more in keeping with that normally used by humans. A third stance, termed unguligrade, involves walking only on the tip of the distal digit. The fact that horses have this posture explains use of the term equinus in DMD patients who toe walk.
DMD and BMD
Most DMD natural history studies include measurements of muscle strength, joint contractures, and timed function tests. Results from these tests are used to track disease progression and offer insight on clinical milestones, such as the loss of ambulation and the need for ventilatory support. Contracture and muscle strength scores generally correlate, deteriorate synchronously over time, and contribute mutually to postural instability. As discussed later, unequal muscle weakness in DMD precipitates a vicious cycle that can lead to debilitating contractures and loss of ambulation ( Fig. 5 ).
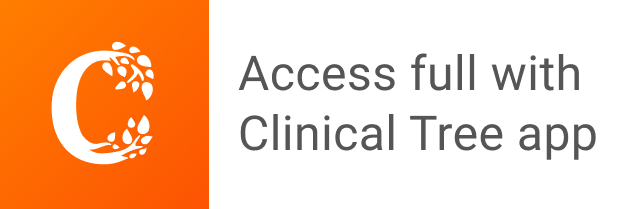